CHAPTER 9 Organization of Motor Function
Movements are the major way that we interact with the world. Most of our activities, whether running, reaching, eating, talking, writing, or reading, ultimately involve motor acts. Thus, motor control is a major task of the nervous system, and from an evolutionary perspective, it is probably the reason that nervous systems first arose. Not surprisingly, a large amount of the nervous system is devoted to motor control, which can be defined as the generation of signals to coordinate contraction of the musculature of the body and head either to maintain a posture or to make a movement (transition between two postures).
Given that large amounts of the nervous system are involved in motor control, it follows that damage or diseases of the nervous system often result in motor abnormalities. Conversely, particular motor symptoms help determine the location of the damaged or malfunctioning region, thus making assessment of motor function an important clinical tool for doctors.
In this chapter each major nervous system area involved in motor control will be described, starting with the spinal cord and then proceeding to the brainstem, cerebral cortex, cerebellum, and basal ganglia. Eye movement will be discussed at the end of the chapter because of the specialized circuits involved in their generation. Although each area will be described separately, it is important to keep in mind that they are highly interdependent and that most movements result from the coordinated action of multiple brain regions. For example, even spinal reflexes, which are mediated by local circuits in the cord, can be modified by descending motor commands, and virtually all voluntary movements are generated by activation of the spinal cord circuitry (or analogous brainstem nuclei for muscles in the head and face).
PRINCIPLES OF SPINAL CORD ORGANIZATION
The spinal cord has several levels of organization, including segmental organization, which will be our initial focus. Segmental organization refers to the fact that there are basic circuits and connections that take place at each level of the spinal cord and that are largely confined to a single or several neighboring segments. The basic spinal reflexes (i.e., the myotactic, inverse myotactic, and flexion reflexes) are mediated by such circuits. However, superimposed on this segmental organization is the propriospinal system, which is a series of neurons whose axons run up and down the spinal cord to connect the different levels of the cord to one another. This system allows the coordination of activity at different spinal levels, which is important for behavior involving forelimbs and hind limbs, such as locomotion. Finally, there are descending motor (and ascending sensory) tracts that interact with these spinal circuits. These motor pathways carry signals related to voluntary movement, but they are also important for the more automatically (or nonconsciously) controlled aspects of motor function, such as the setting of muscle tone (the resting resistance of muscles to changes in length).
Somatic Motor Neurons
Contractions of skeletal muscle fibers are responsible for movement of the body. Skeletal muscle fibers are innervated by large neurons, called αmotor neurons, in the ventral horn of the spinal cord or in cranial nerve nuclei. These neurons are large, multipolar neurons that range in size up to 70 μm in diameter (Fig. 4-10, A). Their axons leave the spinal cord through the ventral roots and from the brainstem via several cranial nerves. The motor axons are distributed to the appropriate skeletal muscles through peripheral nerves, and they terminate with synapses, called neuromuscular junctions or end plates, on skeletal muscle fibers.
A motor unit is an α motor neuron and all of the skeletal muscle fibers that its axon supplies. Each skeletal muscle fiber in mammals is supplied by just one α motor neuron. However, a given α motor neuron may innervate a variable number of skeletal muscle fibers; the number depends on how fine a control of the muscle is required. For highly regulated muscles, such as the eye muscles, an α motor neuron may supply only a few skeletal muscle fibers. However, in a proximal limb muscle, such as the quadriceps femoris, a single α motor neuron may innervate thousands of skeletal muscle fibers.
The motor unit can be regarded as the basic unit of movement. When an α motor neuron discharges under normal circumstances, all the muscle fibers of the motor unit contract. A given α motor neuron may participate in a variety of reflexes and in voluntary movement. Because decisions about whether the synaptic input from various sources will cause particular muscle fibers to contract are made at the level of the α motor neuron (in mammals), these motor neurons have been termed the final common pathway.
Another type of motor neuron is called the γ motor neuron. γ Motor neurons are smaller than α motor neurons; they have a soma diameter of about 35 μm. The γ motor neurons that project to a particular muscle are located in the same motor nucleus as the α motor neurons that supply that muscle. γ Motor neurons do not supply ordinary skeletal muscle fibers. Instead, they synapse on specialized striated muscle fibers, the intrafusal muscle fibers, that are found within muscle spindles (see later).
The skeletal muscle fibers that belong to a given motor unit are called a muscle unit. All the muscle fibers in a muscle unit are of the same histochemical type (i.e., they are all either slow twitch [type I] or fast twitch [type IIA or IIB]). For an in-depth presentation of muscle fiber types, see Chapter 12.
A clinically useful way to monitor the activity of motor units is electromyography. An electrode is placed within a skeletal muscle to record the summed action potentials of the skeletal muscle fibers of a muscle unit (see Fig. 12-7). If no spontaneous activity is noted, the patient is asked to contract the muscle voluntarily to increase the activity of motor units in the muscle. As the force of voluntary contraction increases, more motor units are recruited. In addition to the recruitment of more motor neurons, contractile strength increases with increases in the rate of discharge of the active α motor neurons. Electromyography is used for various purposes. For example, the conduction velocity of motor axons can be estimated by measuring the difference in latency of motor unit potentials when a peripheral nerve is stimulated at two sites separated by a known distance. Another use is to observe fibrillation potentials that occur when muscle fibers are denervated. Fibrillation potentials are spontaneously occurring action potentials in single muscle fibers. These spontaneous potentials contrast with motor unit potentials, which are larger and have a longer duration because they represent the action potentials in a set of muscle fibers that belong to a motor unit.
Spinal Reflexes
A reflex is a relatively predictable, involuntary, and stereotyped response to an eliciting stimulus. Because of these properties, spinal reflexes have been used to identify and classify spinal cord neurons, determine their connectivity, and study their response properties. Thus, knowledge of spinal reflexes is essential for understanding spinal cord function.
The basic circuit that underlies a reflex is called a reflex arc. A reflex arc can be divided into three parts: an afferent limb (sensory receptors and axons) that carries information to the CNS, a central component (synapses and interneurons within the CNS), and an efferent limb (motor neurons) that causes the motor response. The knee jerk response to tapping on the patellar tendon with a reflex hammer by a doctor is a common example of a spinal reflex and illustrates the various components of the definition. The tap on the tendon actually causes brief stretching of the quadriceps muscle (eliciting stimulus) and thus activates sensory receptors (Ia fibers in muscle spindles). Activation of sensory receptors causes an excitatory signal to be sent to the spinal cord to activate motor neurons that go back to the quadriceps and cause it to contract, thereby resulting in a kick (stereotyped response). The person feels the kicking motion but has no sense that it was generated by himself or herself (involuntary). In this case, the afferent limb is represented by the Ia fibers and the efferent limb by the motor neurons. The central portion of this arc is minimal (a synapse from the Ia afferents onto the motor neurons), but in most reflexes it is more complex and can involve multiple types of interneurons.
It is the predictable linking of stimulus and response that makes reflexes a useful tool both for clinicians and for neuroscientists trying to understand spinal cord function. However, one danger to avoid is thinking that a particular neuron’s function is solely participation in a particular reflex because these same neurons are the targets of descending motor pathways and thus are involved in generating voluntary movement. Indeed, many of these neurons are active even when the afferent leg of their reflex arc is silent. One such example is the interneurons of the flexion reflex arc because they are also part of the central pattern generator for locomotion.
Later in this section we will discuss three well-known spinal reflexes because they illustrate important aspects of spinal cord circuitry and function and because of their behavioral and clinical importance. However, you should be aware that there are a number of additional reflexes that are mediated by spinal circuits (e.g., see micturition reflex, Fig. 11-3).
Sensory Receptors Responsible for Eliciting Spinal Reflexes
Each spinal reflex is elicited by the activation of one or more classes of sensory receptors. In the following section, two receptor types, muscle stretch receptors (muscle spindles) and Golgi tendon organs, are described in detail because these receptors are important both for spinal reflexes and as a source of the proprioceptive information that gives us an awareness of our limbs and helps guide voluntary movement.
The Muscle Spindle
Muscle spindles are found in almost all skeletal muscles and are particularly concentrated in muscles that exert fine motor control (e.g., the small muscles of the hand and eye).
Structure of the Muscle Spindle.
As its name implies, a muscle spindle is a spindle or fusiform-shaped organ composed of a bundle of specialized muscle fibers richly innervated both by sensory and by motor axons (Fig. 9-1). A muscle spindle is about 100 μm in diameter and up to 10 mm long. The innervated part of the muscle spindle is encased in a connective tissue capsule. Muscle spindles lie between regular muscle fibers and are typically located near the tendinous insertion of the muscle. The distal ends of the spindle are attached to the connective tissue within the muscle (endomysium). Thus, muscle spindles lie in parallel with the regular muscle fibers. This arrangement has important functional implications, as will be made clear later.
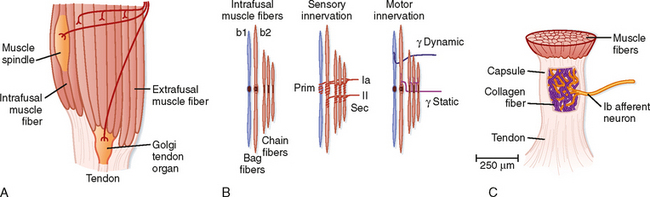
Figure 9-1 Muscle proprioceptors. Skeletal muscles contain sensory receptors embedded within the muscle (spindles) and within their tendons (Golgi tendon organs). A, Schematic of a muscle showing the arrangement of a spindle in parallel with extrafusal muscle fibers and a tendon organ in series with muscle fibers. B, Structure and motor and sensory innervation of a muscle spindle. C, Structure and innervation of a tendon organ.
The muscle fibers within the spindle are called intrafusal fibers to distinguish them from the regular or extrafusal fibers that make up the bulk of the muscle. Individual intrafusal fibers are much narrower than extrafusal fibers and do not run the length of the muscle. Thus, they are too weak to contribute significantly to muscle tension or to directly cause changes in the overall length of the muscle by their contraction.
Morphologically, two types of intrafusal muscle fibers are found within muscle spindles: nuclear bag and nuclear chain fibers (Fig. 9-1, B). These names are derived from the arrangement of nuclei in the fibers. Nuclear bag fibers are larger than nuclear chain fibers, and their nuclei are bunched together like a bag of oranges in the central, or equatorial, region of the fiber. In nuclear chain fibers, the nuclei are arranged in a row. Functionally, nuclear bag fibers are divided into two types: bag1 and bag2. As detailed later, bag2 fibers are functionally similar to chain fibers.
The neural innervation of an intrafusal fiber differs significantly from that of an extrafusal fiber, which is innervated by a single motor neuron. Intrafusal fibers are multiply innervated and receive both sensory and motor innervation. The sensory supply includes a single group Ia afferent and a variable number of group II afferent fibers (Fig. 9-1, B). Group Ia fibers belong to the largest-diameter class of sensory nerve fibers and conduct at 72 to 120 m/sec; group II fibers are intermediate in size and conduct at 36 to 72 m/sec. A group Ia afferent fiber forms a primary ending consisting of a spiral-shaped terminal composed of branches of the group Ia fiber on each of the intrafusal muscle fibers. Thus, terminals of primary endings are found on both types of nuclear bag fibers and on nuclear chain fibers. The group II afferent fiber forms a secondary ending, which is found on nuclear chain and bag2 fibers, but not on bag1 fibers. The primary and secondary endings have mechanosensitive channels that are sensitive to the level of tension on the intrafusal muscle fiber.
The motor supply to a muscle spindle consists of two types of γ motor axons (Fig. 9-1, B). Dynamic γ motor axons end on nuclear bag1 fibers, and static γ motor axons end on nuclear chain and bag2 fibers.
Muscle Spindles Detect Changes in Muscle Length.
Muscle spindles respond to changes in muscle length because they lie in parallel with the extrafusal fibers and therefore will also be stretched or shortened along with the extrafusal fibers. Because intrafusal fibers, like all muscle fibers, display spring-like properties, a change in their length will change the tension that they are under, and this change is sensed by mechanoreceptors of the Ia and II spindle afferents.
Figure 9-2 shows the changes in activity of the afferent fibers of a muscle spindle when the muscle is stretched. It is clear that Ia and II fibers respond differently to stretch. Group Ia fibers are sensitive both to the amount of muscle stretch and to its rate, whereas group II fibers respond chiefly to the amount of stretch. Thus, when a muscle is stretched to a new longer length, group II firing will increase in proportion to the amount of stretch (Fig. 9-2, left), and when the muscle is allowed to shorten, its firing rate will decrease proportionately (Fig. 9-2, right). Group Ia fibers show this same static-type response, and thus under steady-state conditions (i.e., constant muscle length), their firing rate will reflect the amount of muscle stretch, similar to that of group II fibers. However, while muscle length is changing, group Ia firing also reflects the rate of stretch or shortening that the muscle is undergoing. Its activity overshoots during muscle stretch and undershoots (and possibly ceases) during muscle shortening. These are called dynamic responses. This dynamic sensitivity also means that the activity of group Ia fibers is much more sensitive to transient stretches, such as shown in the middle diagrams of Figure 9-2. In particular, the tap profile is what occurs when a doctor uses a reflex hammer to hit the muscle tendon and thereby cause a brief stretching of the attached muscle. The change in muscle length is too brief for changes in group II firing to occur, but because the magnitude of the rate of change (slopes of the tap profile) is so high with this stimulus, large dynamic responses are elicited in the group Ia fibers. Thus, the functionality of reflex arcs involving Ia afferents is what is being assessed by using a reflex hammer to tap on tendons.
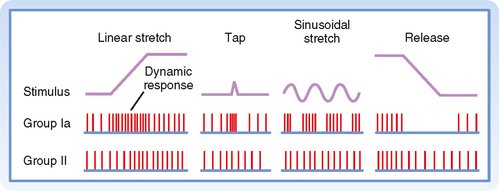
Figure 9-2 Responses of a primary ending (Ia) and a secondary ending (II) to changes in muscle length. Note the difference in dynamic and static responsiveness of these endings. The waveforms at the top represent the changes in muscle length. The middle and bottom rows show the discharges of a group Ia and II fiber, respectively, during the various changes in muscle length.
γ Motor Neurons Adjust the Sensitivity of the Spindle.
Up to this point we have described only how muscle spindles behave when there are no changes in γ motor neuron activity. The efferent innervation of muscle spindles is extremely important, however, because it determines the sensitivity of muscle spindles to stretch. For example, in Figure 9-3, A, the activity of a muscle spindle afferent is shown during a steady stretch. When the extrafusal portion of the muscle contracts (Fig. 9-3, B), the muscle spindle is unloaded by the resultant shortening of the muscle, and the muscle spindle afferent may stop discharging and thus become insensitive to further changes in muscle length. However, this unloading of the spindle can be counteracted if γ motor neurons are simultaneously stimulated. Such stimulation causes the intrafusal muscle fibers of the spindle to shorten along with the extrafusal muscle fibers (Fig. 9-3, C). Actually, only the two polar regions of the intrafusal muscle contract; the equatorial region, where the nuclei are located, does not contract because it has little contractile protein. As a result, when the polar regions contract, the equatorial region elongates and regains its sensitivity. Conversely, when a muscle relaxes and thus elongates, a concurrent decrease in γ motor neuron activity will allow the intrafusal fibers to relax as well and thereby prevent the tension on the central portion of the intrafusal fiber from reaching a level at which firing of the afferents is saturated. Thus, the γ motor neuron system allows the muscle spindle to operate over a wide range of muscle lengths while retaining high sensitivity to small changes in length.
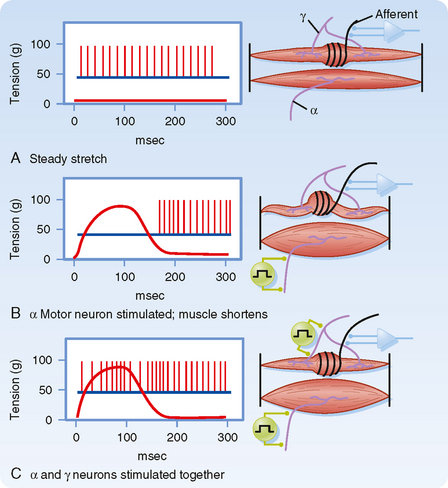
Figure 9-3 The activity of γ motor neurons can counteract the effects of unloading on the discharge of a muscle spindle afferent. A, The activity of a muscle spindle afferent is shown during steady stretch. B, α Motor neuron stimulation at time t = 0 msec causes contraction of the extrafusal fibers, which leads to muscle shortening and increased muscle tension, but unloading of the tension across the muscle spindle, which in turn induces the afferent to stop firing. Upon relaxation the muscle returns to its original length and tension is restored on the intrafusal fibers, causing the return of activity in the Ia afferent. C, Coactivation of α and γ motor neurons causes shortening of both extrafusal and intrafusal fibers. Thus, there is no unloading of the spindle, and the afferent maintains its spontaneous activity.
(Redrawn from Kuffler SW, Nicholls JG: From Neuron to Brain. Sunderland, MA, Sinauer, 1976.)
Descending motor commands from the brain typically activate α and γ motor neurons simultaneously and thus cause a synchronous contraction of extrafusal and intrafusal muscle fibers. This co-contraction means that as the muscle shortens from the contraction of extrafusal fibers, the polar regions of the intrafusal fibers also shorten, thereby maintaining relatively constant tension on the equatorial portion and thus the sensitivity of the spindle apparatus.
As mentioned earlier, there are two types of γ motor neurons—dynamic and static (Fig. 9-1). Dynamic γ motor axons end on nuclear bag1 fibers, and static γ motor axons synapse on nuclear chain and bag2 fibers. Thus, when a dynamic γ motor neuron is activated, the response of the group Ia afferent fiber is enhanced, but the activity of the group II afferents is unchanged; when a static γ motor neuron discharges, the responsiveness of the group II afferents and the static responsiveness of the group Ia afferents are increased. The effects of stimulating the static and dynamic fibers on a group Ia afferent’s response to stretch is illustrated in Figure 9-4. Descending pathways can preferentially influence dynamic or static γ motor neurons and thereby alter the nature of reflex activity in the spinal cord.
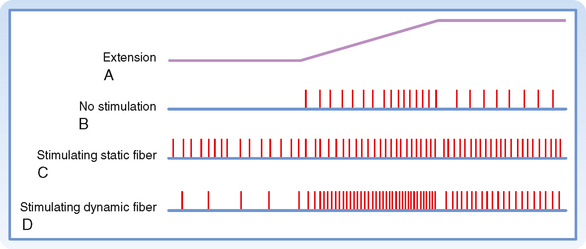
Figure 9-4 Effects of static and dynamic γ motor neurons on the responses of a primary ending to muscle stretch. The upper trace, A, is the time course of the stretch. B shows the discharge of group Ia fibers in the absence of γ motor neuron activity. In C, a static γ motor axon was stimulated, and in D, a dynamic γ motor axon was stimulated.
(Redrawn from Crowe A, Matthews PBC: J Physiol 174:109, 1964.)
Golgi Tendon Organ
A second type of mechanosensitive receptor associated with skeletal muscle is the Golgi tendon organ (Fig. 9-1). A Golgi tendon organ is innervated from the terminals of group Ib afferent fibers. The diameter of a Golgi tendon organ is about 100 μm and its length is about 1 mm. A group Ib fiber has a large diameter and conducts in the same velocity range as a group Ia fiber. The terminals of a Ib fiber are wrapped about bundles of collagen fibers in the tendon of a muscle (or in tendinous inscriptions within the muscle). Thus, the sensory ending is arranged in series with the muscle, in contrast to the parallel arrangement of the muscle spindle.
The distinction between the responsiveness of the muscle spindles and Golgi tendon organs can be made clear by comparing the firing patterns of Ia and Ib fibers when a muscle is stretched and then held at a longer length (Fig. 9-5). The Ia fiber’s firing rate will maintain its increase until the stretch is reversed. In contrast, the Ib fiber will show an initial large increase in firing, reflecting the increased tension on the muscle caused by the stretch, but will then show a gradual return toward its initial firing rate as the tension on the muscle is lowered because of cross-bridge recycling and the resultant lengthening of the sarcomeres. Therefore, Golgi tendon organs signal force, whereas spindles signal muscle length. Further evidence of this distinction is that Ib firing correlates with force level during isometric contraction even though muscle length, and therefore Ia activity, are unchanged.
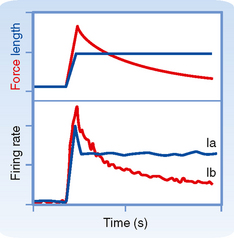
Figure 9-5 Changes in group Ia and Ib firing rates when muscle is stretched to a new length as indicated in the top graph (blue line). After a transient burst, the firing rate of the Ia fiber remains constant at a new higher level that is proportional to the increase in length (lower graph, blue line). In contrast, the Ib unit shows an initial rapid increase in firing followed by a slow decrease back toward its original level (bottom graph, red line) and has a firing profile that matches the tension level in the muscle caused by the stretch (top graph, red line).
The Myotatic or Stretch Reflex
The stretch reflex is key for the maintenance of posture and helps overcome unexpected impediments during a voluntary movement. Changes in the stretch reflex are involved in actions commanded by the brain, and pathological alterations in this reflex are important signs of neurological disease. The phasic stretch reflex occurs in response to rapid, transient stretches of the muscle, such as those elicited by a doctor using a reflex hammer or by an unexpected impediment to an ongoing movement. The tonic stretch reflex occurs in response to a slower or steady stretch applied to the muscle.
The Phasic (or Ia) Stretch Reflex.
The phasic stretch reflex is elicited by the primary endings of the muscle spindles. The reflex arc responsible for the phasic stretch reflex is shown in Figure 9-6. A group Ia afferent fiber from a muscle spindle in the rectus femoris muscle is shown to branch as it enters the gray matter of the spinal cord. It will form excitatory synapses directly (monosynaptically) on virtually all α motor neurons that supply the same (also known as the homonymous) muscle and with many of its synergists, such as the vastus intermedius muscle in this case, which also acts to extend the leg at the knee. If the excitation is powerful enough, the motor neurons discharge and cause a contraction of the muscle. Note that the Ia fibers do not contact the γ motor neurons, possibly to avoid a positive-feedback loop situation. This selective targeting of α motor neurons is exceptional in that most other reflex and descending pathways will target both α and γ motor neurons.
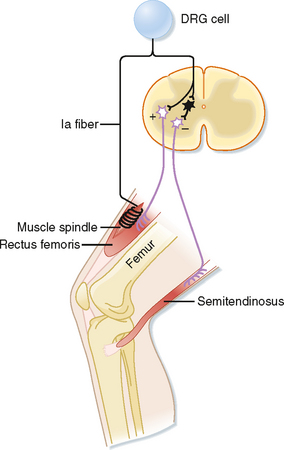
Figure 9-6 Reflex arc of the stretch reflex. The shortest pathway in this arc contains a single synapse within the CNS; hence, it is a monosynaptic reflex. The interneuron, shown in black, is a group Ia inhibitory interneuron.
Other branches of group Ia fibers end on a variety of interneurons; however, one type, the reciprocal Ia inhibitory interneuron (black cell in Fig. 9-6), is particularly important with regard to the stretch reflex. These interneurons are identifiable because they are the only inhibitory interneurons that receive input from both the Ia afferents and Renshaw cells (see Fig. 9-11). They end on α motor neurons that innervate the antagonist muscles, in this case the hamstring muscles, including the semitendinosus muscle, which act to flex the knee.
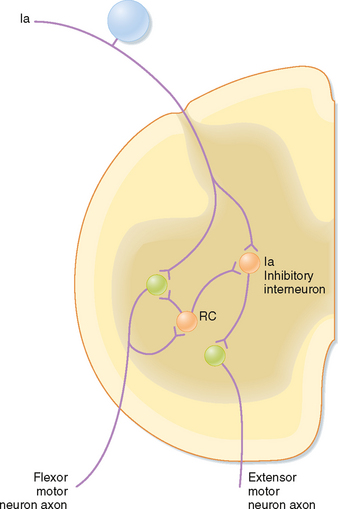
Figure 9-11 Renshaw cell (RC) connections with motor neurons and Ia inhibitory interneurons. The circuits shown mediate Ia reciprocal inhibition of antagonist muscles (in this case an extensor) and inhibition of this reciprocal inhibition by Renshaw cells. Note that there are equivalent Renshaw cells and Ia inhibitory interneurons associated with extensor motor neurons and Ia input from spindles in extensor muscles, but they are not shown for simplicity. Orange cells are inhibitory and blue and green ones are excitatory.
The organization of the stretch reflex arc guarantees that one set of α motor neurons is activated and the opposing set is inhibited. This arrangement is known as reciprocal innervation. Although many reflexes involve such reciprocal innervation, this type of innervation is not the only possible organization of a motor control system, and indeed, descending motor pathways can override such patterns.
The stretch reflex is quite powerful, in large part because of its monosynaptic nature. The power of this reflex also derives from the essentially maximal convergence and divergence that exist in this pathway, which is not apparent from the circuit diagrams, such as Figure 9-6, that are typically used to illustrate reflex pathways. That is, each Ia fiber will contact virtually all homonymous α motor neurons, and each such α motor neuron will receive input from every spindle in that muscle. Although its monosynaptic nature makes the Ia reflex rapid and powerful, it also means that there is relatively little opportunity for direct control of activity flow through its reflex arc. The CNS overcomes this problem by controlling muscle spindle sensitivity via the γ motor neuron system.
The Tonic Stretch Reflex.
The tonic stretch reflex can be elicited by passively bending a joint. This reflex circuit includes both group Ia and group II afferent fibers from muscle spindles. Group II fibers make monosynaptic excitatory connections with α motor neurons, but they also excite them through disynaptic and polysynaptic pathways. Normally, there is ongoing activity in the Ia and II afferents that helps maintain a baseline firing of α motor neurons; therefore, the tonic stretch reflex contributes to muscle tone. Its activity also contributes to our ability to maintain a posture. For example, if the knee of a soldier standing at attention begins to flex because of fatigue, the quadriceps muscle will be stretched, a tonic stretch reflex will be elicited, and the quadriceps will contract more, thereby opposing the flexion and restoring the posture.
Hyperactive stretch reflexes can lead to tremors and clonus. Although the negative-feedback action of the stretch reflex should help stabilize the limb, the conduction delay between the initiating stimulus (muscle stretch) and the response (muscle contraction) can cause it to be a source of instability resulting in rhythmic movements such as tremors and clonus. Clonus is elicited by a sustained stretch of a muscle in a person who has spinal cord damage. Normally, an imposed sustained stretch on a muscle will elicit an increase in Ia and II activity, which after a delay will cause a contraction in the muscle that opposes the stretch but does not completely return the muscle to its initial length because the gain of the stretch reflex is much less than 1.* This partial compensation, in turn, will lead to a decrease in Ia and II activity, which causes the limb to lengthen again, but not fully. This lengthening will once again increase Ia and II activity, and so on. The delay is key in setting up this oscillation because it leads to the feedback signal continuing even after the muscle has compensated and thus results in an overcompensation that leads to the next overcorrection. However, because the reflex gain is normally much less than 1, this oscillation dies out quickly (the overcompensation gets smaller and smaller), and the muscle comes to rest at an intermediate length. In contrast, when descending motor pathways are damaged, the resulting changes in spinal cord connectivity and increases in neuronal excitability result in a hyperactive reflex (which is equivalent to raising the gain close to 1). In this case, the successive overcompensations are much larger, and an overt but transient oscillation can be observed (clonus). If the gain equals 1, the clonus does not die out but rather persists for as long as the initial stretch stimulus is maintained.
The foregoing discussion suggests that stretch reflexes can act like a negative-feedback system to control muscle length. By following the stretch reflex arc, it is possible to see that changes in its activity will act to oppose changes in muscle length from a particular equilibrium point. For example, if the muscle’s length is increased, there will be an increase in Ia and II firing, which will excite homonymous α motor neurons and lead to contraction of the muscle and reversal of the stretch. Similarly, passive shortening of the muscle will unload the spindles and lead to a decrease in the excitatory drive to the motor neurons and thus relaxation of the muscle. So how are we able to rotate our joints? It is partly because the γ motor neurons are coactivated during a movement and thereby shift the equilibrium point of the spindle and partly because the gain or strength of the reflex is low enough that other input to the motor neuron can override the stretch reflex.
Inverse Myotatic or Ib Reflex
Just as the stretch reflex can be thought of as a feedback system to regulate muscle length, the inverse myotactic, or Ib, reflex can be thought of as a feedback system to help maintain force levels in a muscle. Using the upper part of the leg as an example, the Ib reflex arc is shown in Figure 9-7. In this example the receptor organs are Golgi tendon organs of the rectus femoris muscle. The afferent fibers branch as they enter the spinal cord and end on interneurons. There are no monosynaptic connections to α motor neurons. Rather, the Ib afferents synapse onto two classes of interneurons: interneurons that inhibit α motor neurons that supply the homonymous muscle, in this case the rectus femoris, and excitatory interneurons that activate α motor neurons to the antagonist (semitendinosus). Because there are two synapses in series in the CNS, this is a disynaptic reflex arc. Given these connections, Ib activity should have the opposite action of the Ia stretch reflex during passive stretch of the muscle, which explains this reflex’s other name, the inverse myotactic reflex. However, functionally, the two reflex arcs can act synergistically, as the following example shows. Recall that the Golgi tendon organs monitor force levels across the tendon that they supply. If during maintained posture, such as standing at attention, knee extensors, such as the rectus femoris muscle, begin to fatigue, the force in the patellar tendon will decline. The decline in force will reduce the activity of Golgi tendon organs in this tendon. Because the Ib reflex normally inhibits the α motor neurons to the rectus femoris muscle, reduced activity of the Golgi tendon organs will enhance the excitability of (i.e., disinhibit) the α motor neurons and thereby help reverse the decrease in force caused by the fatigue. Simultaneously, bending of the knee will stretch the knee extensors and activate the Ia fibers, which will then excite the same α motor neurons. Thus, coordinated action of both muscle spindle and Golgi tendon organ afferent fibers is needed to cause greater contraction of the rectus femoris muscle and maintenance of the posture.
Flexion Reflexes and Locomotion
The flexion reflex starts with activation of one or more of a variety of sensory receptors, including nociceptors, whose signals can be carried to the spinal cord via a variety of afferents, including group II and III fibers, collectively called the flexion reflex afferents (FRAs). In flexion reflexes, afferent volleys (1) cause excitatory interneurons to activate the α motor neurons that supply the flexor muscles in the ipsilateral limb and (2) cause inhibitory interneurons to inhibit the α motor neurons that supply the antagonistic extensor muscles (Fig. 9-8). This pattern of activity causes one or more joints in the stimulated limb to flex. In addition, commissural interneurons evoke the opposite pattern of activity in the contralateral side of the spinal cord (Fig. 9-8), which results in extension of the opposite limb, the crossed extension reflex. For our lower limbs (or in quadrupeds for both forelimbs and hind limbs), the crossed extension part of the reflex helps in maintaining balance by enabling the contralateral limb to be able to support the additional load that is transferred to it when the flexed limb is lifted.
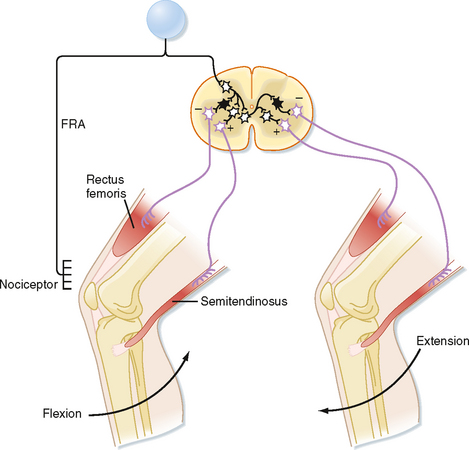
Figure 9-8 The reflex arc of the flexion reflex. Black interneurons are inhibitory and clear ones are excitatory. FRA, flexion reflex afferent.
After damage to the descending motor pathways, hyperactive stretch reflexes may result in spasticity, in which there is large resistance to passive rotation of the limbs. In this condition it may be possible to demonstrate what is called the clasp-knife reflex. When spasticity is present, attempts to rotate a limb about a joint will initially meet high resistance. However, if the applied force is increased, there will come a point at which the resistance suddenly dissipates and the limb rotates easily. This change in resistance is caused by reflex inhibition. The Ib reflex arc suggests that rising activity in this pathway could underlie the sudden release of resistance, and indeed, the clasp-knife reflex was once attributed to the activation of Golgi tendon organs when these receptors were thought to have a high threshold to muscle stretch. However, the tendon organs have since been shown to be activated at very low levels of force and are no longer thought to cause the clasp-knife reflex. It is now thought that this reflex is caused by the activation of other high-threshold muscle receptors that supply the fascia around the muscle. Signals from these receptors cause the activation of interneurons that lead to inhibition of the homonymous motor neurons.
Because flexion typically brings the affected limb in closer to the body and away from a painful stimulus, flexion reflexes are a type of withdrawal reflex. In Figure 9-8, the neural circuit of the flexion reflex is shown for neurons that affect only the knee joint. Actually, however, considerable divergence of the primary afferent and interneuronal pathways occurs in the flexion reflex. In fact, all the major joints of a limb (e.g., hip, knee, and ankle) may be involved in a strong flexor withdrawal reflex. Details of the flexor withdrawal reflex vary, depending on the nature and location of the stimulus. This variability in flexion reflex is called the local sign. Flexor withdrawal reflexes also occur in areas other than the limbs; for example, visceral disease may cause contractions of muscles in the chest wall or abdomen and thereby decrease the mobility of the trunk.
To show that these circuits are actually involved in generating the locomotion rhythm, spinal cord preparations were made that showed spontaneous locomotion (i.e., if the brainstem is transected and weight is supported, the spinal cord circuits can generate activity that causes the limbs to generate a normal locomotion sequence.) In one such preparation, the electromyogram from the flexors and extensors of a limb were recorded and the FRAs then stimulated to see the effect on locomotion rhythm (Fig. 9-9). Before any stimulus, a spontaneous alternating pattern of flexor and extensor electromyographic (EMG) activity exists. If the FRAs were not involved in the locomotion circuit or at least were not a critical part of the circuits responsible for generating the rhythm (Fig. 9-9, B), we would expect the stimulus to produce only a transient response (i.e., a single EMG response of the flexors and brief inhibition of the extensors) and have no long-term effect on this pattern. Such a transient response is observed (Fig. 9-9, A; EMG records just after the stimulus). However, the stimulus also causes a permanent, approximately 180-degree phase shift in locomotor rhythm, as can be seen by comparing the times of contractions before and after the stimulus. The dashed vertical lines indicate the times at which a flexor EMG response would be expected if the stimulus had produced no phase shift from the EMG activity pattern; before the stimulus, each vertical line is aligned with the onset of a flexor EMG burst, whereas after the stimulus, each vertical line occurs at the end of the flexor burst. Therefore, we can conclude that the stimulus affected the locomotor CPG itself and that the FRA interneurons are a critical part of this CPG (Fig. 9-9, C).
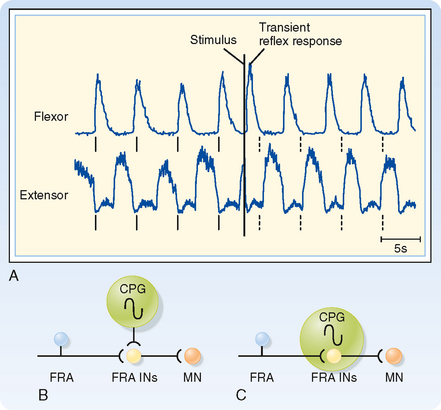
Figure 9-9 Phase reset of locomotion rhythm by FRA stimulation helps identify neuronal components of the underlying central pattern generator (CPG). A, EMG records from knee flexor and extensor muscles. Note the rhythmic alternating pattern before application of the stimulus. The solid vertical lines below each trace indicate the times at which flexor contraction is initiated. The dashed vertical lines indicate the times at which flexor contraction would have been initiated if the stimulus caused no lasting effect on the rhythmic pattern. B and C, Two possible models for the CPG underlying the locomotor rhythm seen in A. B does not include the FRA interneurons in the CPG, whereas C does. The data shown in A support the model shown in C.
(Data from Hultborn H et al: Ann N Y Acad Sci 860:70, 1998.)
A second important point illustrated by this experiment is that the locomotion CPG (and CPGs generally) can be influenced by strong afferent activity. The afferent influence ensures that the pattern generator adapts to changes in the terrain as locomotion proceeds. Such changes may occur rapidly during running, and locomotion must then be adjusted to ensure proper coordination.
Determining Spinal Cord Organization by Using Reflexes
As already discussed, divergence is an important aspect of reflex pathways. Convergence is another important organizational feature of reflex arcs. Convergence is defined as the termination of several neurons on one other neuron. For example, all group Ia afferent fibers from the muscle spindles of a particular hind limb muscle synapse onto any given α motor neuron to that muscle. Convergent input can be demonstrated by using the phenomenon of spatial facilitation, which is illustrated in Figure 9-10.
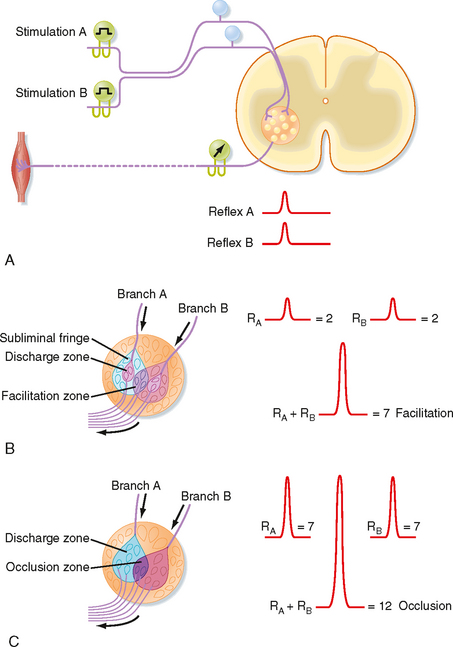
Figure 9-10 A, Arrangement for using electrically evoked afferent volleys and recordings from motor axons in a ventral root to study reflexes. B, Experiment in which combined stimulation of two muscle nerves resulted in spatial summation. In C, the combined volleys caused occlusion.
(Redrawn from Eyzaguirre C, Fidone SJ: Physiology of the Nervous System, 2nd ed. Chicago, Mosby—Year Book, 1975.)
In this example, a monosynaptic reflex is elicited by electrical stimulation of the group Ia fibers in each of two branches of a muscle nerve (Fig. 9-10, A). The reflex response is characterized by recording the discharges of α motor axons from the appropriate ventral root (as a compound action potential). When muscle nerve branch A is stimulated, a small compound action potential is recorded as reflex A. Similarly, when muscle nerve branch B is stimulated, reflex B is recorded. Figure 9-10, B, depicts the motor neurons contained within the motor nucleus. The discharge zones (pink colored areas) enclose α motor neurons that are activated above threshold when each muscle nerve branch is stimulated separately. Thus, two α motor neurons spike when each muscle nerve branch is stimulated alone (an additional seven motor neurons in the subliminal fringe are excited, but not sufficiently to trigger spikes). When the two nerves are stimulated at the same time, a much larger reflex discharge is recorded (see recordings at the right of Fig. 9-10, B). As the figure demonstrates, this reflex represents the discharge of seven α motor neurons: the four that spiked after the singular stimulation of each nerve (two per nerve) and three additional α motor neurons (located in the facilitation zone) that are made to discharge only when the two muscle nerves are stimulated simultaneously because they lie in the subliminal fringe for both nerves.
A similar effect could be elicited by repetitive stimulation of one of the muscle nerves, provided that the stimuli occur close enough together that some of the excitatory effect of the first volley still persists after the second volley arrives. This effect is called temporal summation. Both spatial summation and temporal summation depend on the properties of the EPSPs evoked in α motor neurons by the group Ia afferent fibers (see Fig. 6-8).
If a volley in one of the two muscle nerves in Figure 9-10 reaches the motor nucleus at a time when the motor neurons are highly excitable, the reflex discharge will be relatively large (see Fig. 9-10, C). A similar volley in the other muscle nerve might also produce a large reflex response. However, when the two muscle nerves are excited simultaneously, the reflex can be less than the sum of the two independently evoked reflexes if the cells reaching threshold to activation of either of the two nerves alone overlap significantly. In this case, each afferent nerve activates 7 α motor neurons, but the volleys in the two nerves together cause only 12 motor neurons to discharge. This phenomenon is called occlusion.
The phenomena of spatial and temporal summation and occlusion can also be used to demonstrate interactions between spinal cord neurons and the various reflex circuits. To start, a monosynaptic reflex discharge can be evoked by stimulating the group Ia afferent fibers in a muscle nerve. This tests the reflex excitability of a population of α motor neurons. The discharges of either extensor or flexor α motor neurons can be recorded by choosing the proper muscle nerve to be stimulated. Other kinds of afferent fibers are then stimulated along with the homonymous Ia afferent from the muscle to see whether the response to the Ia stimulation changes. For example, stimulation of group Ia afferent fibers in the nerve to the antagonist muscles produces inhibition of the response to the homonymous Ia stimulation (which is mediated by what is called the reciprocal Ia inhibitory interneuron). Alternatively, if the small afferent fibers of a cutaneous nerve are stimulated to evoke a flexion reflex, the responses to Ia stimulation of the α motor neurons that innervate the extensor muscles will be inhibited (and those of α motor neurons that innervate flexor muscles will be potentiated). As a final example, stimulation of a ventral root causes inhibition of Ia responses and inhibits the reciprocal Ia inhibition. Because the ventral root contains only motor neuron axons, this result implies the presence of axon collaterals that excite inhibitory interneurons that feed back onto the same motor neuron population (Fig. 9-11). These interneurons are named Renshaw cells. Because ventral root stimulation also inhibits the Ia inhibition of antagonist motor neurons, but no other classes of interneurons, the reciprocal Ia interneurons can be uniquely identified by their being inhibited by ventral root stimulation (and activated by Ia stimulation).
Topographic Organization of the Ventral Horn
Up to this point we have considered the functional organization of the spinal cord, largely without regard to its physical (i.e., anatomic) instantiation. We now turn to this aspect of spinal cord organization by discussing the organization of the ventral horn and in particular the topographic arrangement of the motor neurons contained therein. This topography has functional implications for how the descending motor tracts interact with the spinal cord machinery that we have been discussing.
Spinal cord motor neurons are organized topographically in rostrocaudally running columns in the ventral horn (Fig. 9-12). Motor neurons that supply the axial musculature form a column of cells that extends the length of the spinal cord. In the cervical and lumbosacral enlargements, these cells are located in the most medial part of the ventral horn. Motor neurons that supply the limb muscles form columns that extend for several segments in the lateral part of the ventral horn in the cervical and lumbosacral enlargements. Motor neurons to muscles of the distal part of the limb are located most laterally, whereas those that innervate more proximal muscles are located more medially. Motor neurons to flexors are dorsal to those that innervate extensors. Note that the α and γ motor neurons to a given muscle are found intermixed within the same motor neuron column.
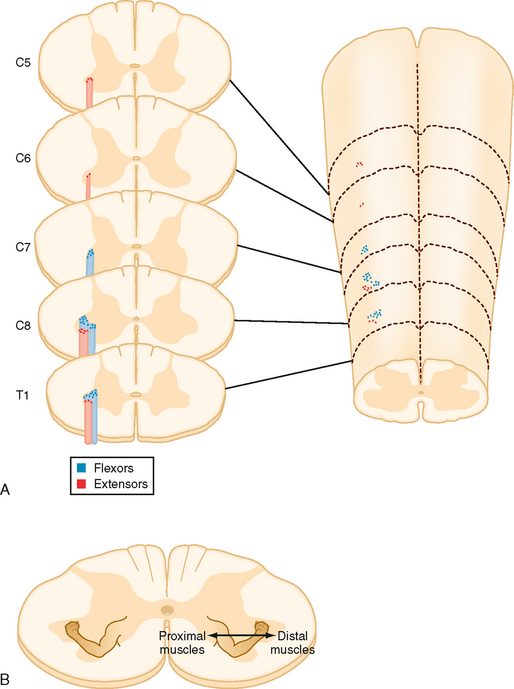
Figure 9-12 Musculotopic organization of motor neurons in the ventral horn of the spinal cord. A, Schematic of the cervicothoracic spinal cord and associated cross sections, showing the locations of motor neurons that innervate a flexor (blue dots) and an extensor (red dots). B, Spinal cord cross section with locations of different muscles represented by a drawing of the arm.
(Redrawn from Purves D et al [eds]: Neuroscience, 3rd ed. Sunderland, MA, Sinauer, 2004.)
The interneurons that connect with the motor neurons in the enlargements are also topographically organized. In general, interneurons that supply the limb muscles are located mainly in the lateral parts of the deep dorsal horn and the intermediate region that lies between the dorsal and ventral horns. Those that supply the axial muscles, however, are located in the medial part of the ventral horn. These interneurons receive synaptic connections from primary afferent fibers and from the axons of pathways that descend from the brain, and thus are both part of spinal reflex arcs and descending motor control pathways.
An important aspect of interneuronal systems is that the laterally placed interneurons project ipsilaterally to motor neurons that supply the distal or the proximal limb muscles, whereas the medial interneurons project bilaterally. This arrangement of the lateral interneurons allows the limbs to be controlled independently. In contrast, the bilateral arrangement of the medial interneurons allows bilateral control of motor neurons to the axial muscles to provide postural support to the trunk and neck.
DESCENDING MOTOR PATHWAYS
Classification of Descending Motor Pathways
Pyramidal versus Extrapyramidal Pathways
Descending motor pathways were traditionally subdivided into pyramidal tract and extrapyramidal pathways. This terminology reflects a clinical dichotomy between pyramidal tract disease and extrapyramidal disease. In pyramidal tract disease, the corticospinal, or pyramidal, tract is interrupted. The signs of this disease were originally attributed to the loss of function of the pyramidal tract (so named because the corticospinal tract passes through the medullary pyramid). However, in many cases of pyramidal tract disease, the functions of other pathways are also altered, and most pyramidal tract signs (see the later section Motor Deficits Caused by Lesions of Descending Motor Pathways) appear to not be caused by loss of the corticospinal tract or at least require damage to additional motor pathways. The term extrapyramidal is even more problematic. Thus, this classification system is not used in this book.
Lateral versus Medial Motor Systems
Another way of classifying the motor pathways is based on their sites of termination in the spinal cord and the consequent differences in their roles in the control of movement and posture. The lateral pathways terminate in the lateral portions of the spinal cord gray matter (Fig. 9-13). The lateral pathways can excite motor neurons directly, although interneurons are their main target. They influence reflex arcs that control fine movement of the distal ends of limbs, as well as those that activate supporting musculature in the proximal ends of limbs. The medial pathways end in the medial ventral horn on the medial group of interneurons (Fig. 9-13). These interneurons connect bilaterally with motor neurons that control the axial musculature and thereby contribute to balance and posture. They also contribute to the control of proximal limb muscles. In this book we use the lateral/medial terminology to classify the descending motor pathways. However, even this scheme is not perfect, partly because although motor neuron cell bodies form localized columns, motor neuron dendritic trees are rather large and typically span most of the ventral horn. Thus, any motor neuron can potentially receive input from so-called medial or lateral system pathways.
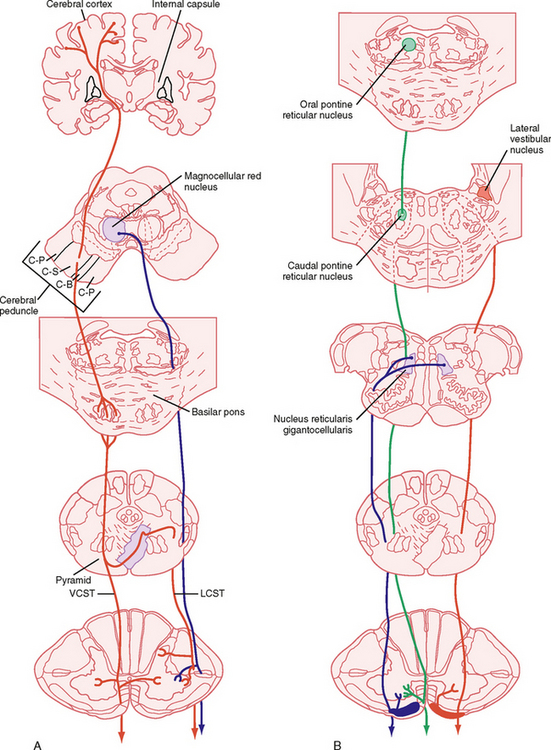
Figure 9-13 Descending motor pathways. Major pathways connecting the cortical and brainstem motor areas to the spinal cord are shown. A, Lateral system pathways, corticospinal (red) and rubrospinal (blue) pathways. Note that the ventral corticospinal pathway is part of the medial system, but is shown in A for simplicity. B, Medial system pathways, medullary (blue) and pontine (green) reticulospinal and lateral vestibulospinal (red) pathways. C-B, corticobulbar; C-P, corticopontine; C-S, corticospinal; LCST, lateral corticospinal tract; VCST, ventral corticospinal tract.
The Lateral System
Lateral Corticospinal and Corticobulbar Tracts
The corticospinal and corticobulbar tracts originate from a wide region of the cerebral cortex. This region includes the primary motor, premotor, supplementary, and cingulate motor areas of the frontal lobe and the somatosensory cortex of the parietal lobe. The cells of origin of these tracts include both large and small pyramidal cells of layer V of the cortex, including the giant pyramidal cells of Betz. Although Betz cells are a defining feature of the motor cortex, they represent a small minority (< 5%) of the cells that contribute to these tracts, in part because they are found only in the primary motor cortex, and even here they represent a minority of the cells contributing to the tract. These tracts leave the cortex and enter the internal capsule, then traverse the midbrain in the cerebral peduncle, pass through the basilar pons, and emerge as the pyramids on the ventral surface of the medulla (Fig. 9-13, A). The corticobulbar axons leave the tract as it descends the brainstem and terminate in the various cranial nerve motor nuclei. The corticospinal fibers continue caudally, and in the most caudal region of the medulla, about 90% of them cross to the opposite side. They then descend in the contralateral lateral funiculus as the lateral corticospinal tract. The lateral corticospinal axons terminate at all spinal cord levels, primarily on interneurons, but also on motor neurons. The remaining uncrossed axons continue caudally in the ventral funiculus on the same side as the ventral corticospinal tract, which belongs to the medial system. Many of these fibers ultimately decussate at the spinal cord level at which they terminate.
The corticobulbar tract, which projects to the cranial nerve motor nuclei, has subdivisions that are comparable to the lateral and ventral corticospinal tracts. For example, part of the corticobulbar tract ends contralaterally in the portion of the facial nucleus that supplies muscles of the lower part of the face and in the hypoglossal nucleus. This component of the corticobulbar tract is organized like the lateral corticospinal tract. The remainder of the corticobulbar tract ends bilaterally.
Rubrospinal Tract
This tract originates in the magnocellular portion of the red nucleus, which is located in the midbrain tegmentum. These fibers decussate (cross) in the midbrain, descend through the pons and medulla, and then take up a position just ventral to the lateral corticospinal tract in the spinal cord. They preferentially affect motor neurons controlling distal musculature, similar to the corticospinal fibers. Red nucleus neurons receive input from the cerebellum and from the motor cortex, thus making this an area of integration of activity from these two motor systems.
The Medial System
The ventral corticospinal tract and much of the corticobulbar tract can be regarded as medial system pathways. These tracts end on the medial group of interneurons in the spinal cord and on equivalent neurons in the brainstem. The axial muscles are controlled by these pathways. These muscles often contract bilaterally to provide postural support or some other bilateral function, such as swallowing or wrinkling of the brow.
Other medial system pathways originate in the brainstem. These include the pontine and medullary reticulospinal tracts, the lateral and medial vestibulospinal tracts, and the tectospinal tract.
Pontine and Medullary Reticulospinal Tracts
The cells that give rise to the pontine reticulospinal tract are in the medial pontine reticular formation. The tract descends in the ventral funiculus, and it ends on the ipsilateral medial group of interneurons. Its function is to excite motor neurons to the proximal extensor muscles to support posture.
The medullary reticulospinal tracts arise from neurons of the medial medulla, in particular the nucleus gigantocellularis. The tracts descend bilaterally in the ventral lateral funiculus, and they end mainly on interneurons associated with medial motor neuron cell groups. The function of the pathway is mainly inhibitory.
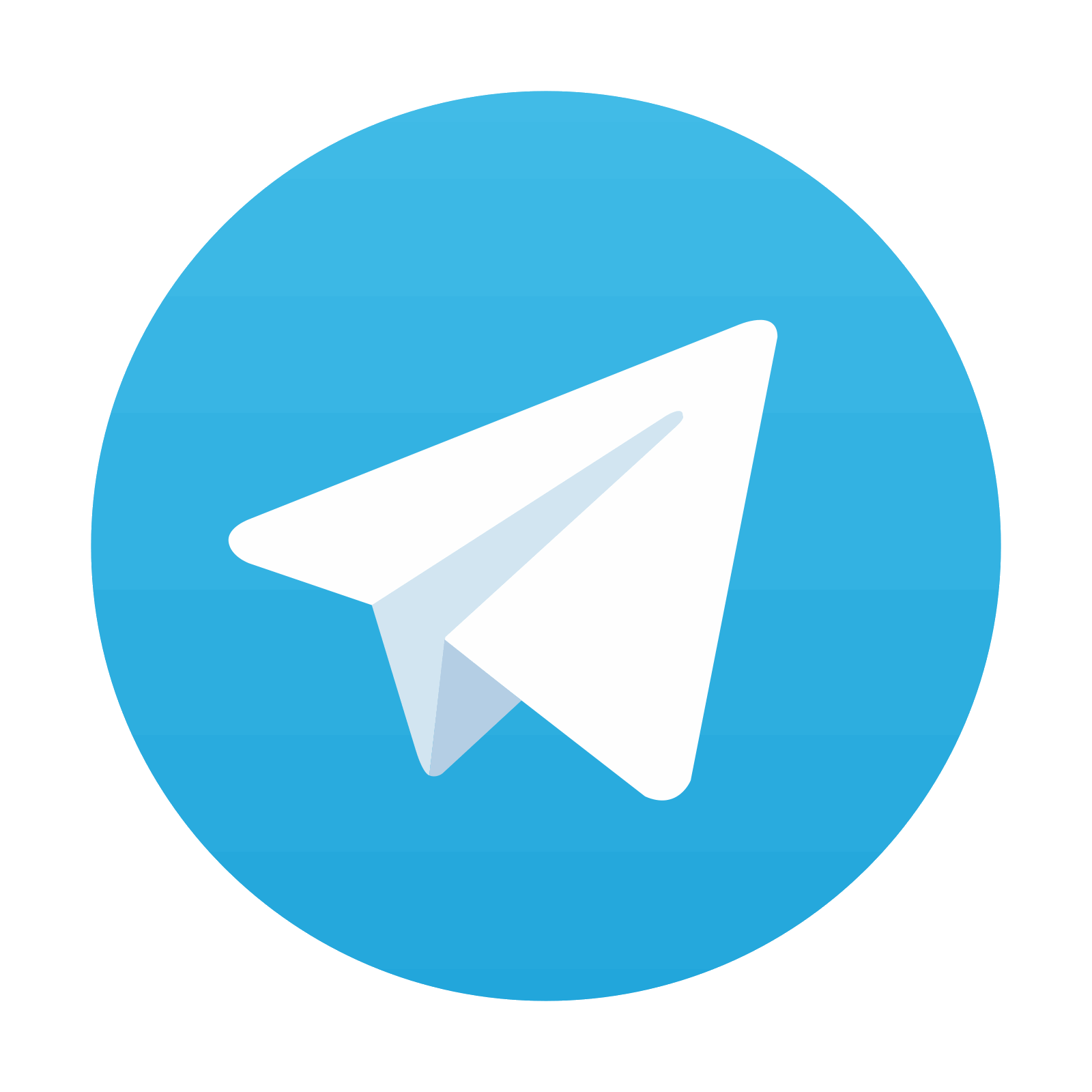
Stay updated, free articles. Join our Telegram channel
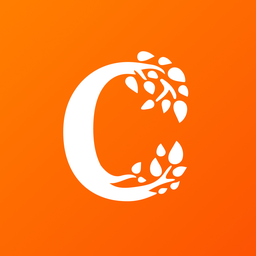
Full access? Get Clinical Tree
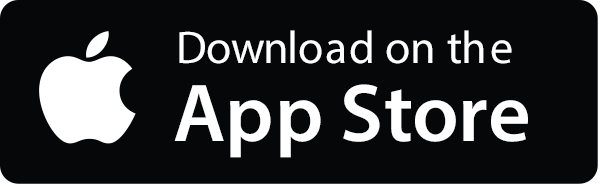
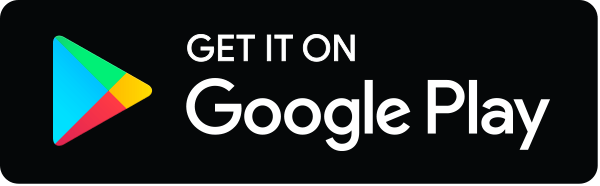