OBJECTIVES
After studying this chapter, youshould be able to:
List the major types of neurotransmitters.
Summarize the steps involved in the biosynthesis, release, action, and removal from the synaptic cleft of the major neurotransmitters.
Describe the various types of receptors for amino acids, acetylcholine, monoamines, ATP, opioids, nitric oxide, and cannabinoids.
Identify the endogenous opioid peptides, their receptors, and their functions.
INTRODUCTION
Nerve endings have been called biologic transducers that convert electrical energy into chemical energy. An observation made by Otto Loewi, a German pharmacologist, serves as the foundation for the concept of chemical neurotransmission and his receipt of the Nobel Prize in Physiology and Medicine in 1936. He provided the first decisive evidence that a chemical messenger was released by the vagus nerve supplying the heart to reduce heart rate. The experimental design came to him in a dream on Easter Sunday of 1920. He awoke from the dream, jotted down notes, but the next morning they were indecipherable. The next night, the dream recurred and he went to his laboratory at 3:00 AM to conduct a simple experiment on a frog heart. He isolated the hearts from two frogs, one with and one without its innervation. Both hearts were attached to cannulas filled with a saline solution. The vagus nerve of the first heart was stimulated, and then the saline solution from that heart was transferred to the noninnervated heart. The rate of its contractions slowed as if its vagus nerve had been stimulated. Loewi called the chemical released by the vagus nerve vagusstoff. Not long after, it was identified chemically to be acetylcholine. Loewi also showed that when the sympathetic nerve of the first heart was stimulated and its effluent was passed to the second heart, the rate of contraction of the “donor” heart increased as if its sympathetic fibers had been stimulated. These results proved that nerve terminals release chemicals that cause the modifications of cardiac function that occur in response to stimulation of its nerve supply.
CHEMICAL TRANSMISSION OF SYNAPTIC ACTIVITY
Regardless of the type of chemical mediator involved, several common steps comprise the process of transmission at a chemical synapse. The first steps are the synthesis of the neurotransmitter usually within the nerve terminal and its storage within synaptic vesicles. This is followed by release of the chemical into the synaptic cleft in response to nerve impulses. The secreted neurotransmitter can then act on receptors on the membrane of the postsynaptic neuron, effector organ (eg, muscle or gland), or even on the presynaptic nerveterminal. Thefinal steps in the process lead to termination of the actions of the neurotransmitter and include diffusion away from the synaptic cleft, reuptake into the nerve terminal, and enzymatic degradation. All of these processes, plus the events in the postsynaptic neuron, are regulated by many physiologic factors and can be altered by drugs. Therefore, pharmacologists (in theory) should be able to develop drugs that regulate not only somatic and visceral motor activity but also emotions, behavior, and all the other complex functions of the brain. Some chemicals released by neurons have little or no direct effects on their own but can modify the effects of neurotransmitters. These chemicals are called neuromodulators.
Many neurotransmitters and the enzymes involved in their synthesis and catabolism have been localized in nerve endings by immunohistochemistry, a technique in which antibodies to a given substance are labeled and applied to the brain and other tissues. The antibodies bind to the substance, and the location of the substance is then determined by locating the label with the light or electron microscope. In situ hybridization histochemistry, which permits localization of the mRNAs for particular synthesizing enzymes or receptors, has also been a valuable tool.
There are two main classes of chemical substances that serve as neurotransmitters and neuromodulators: small-molecule transmitters and large-molecule transmitters. Small-molecule transmitters include amino acids (eg, glutamate, γ-aminobutyric acid [GABA], and glycine), acetylcholine, monoamines (eg, norepinephrine, epinephrine, dopamine, and serotonin), and adenosine triphosphate (ATP). Large-molecule transmitters include neuropeptides such as substance P, enkephalin, vasopressin, and a host of others. In general, neuropeptides are co-localized with one of the small-molecule neurotransmitters (Table 7–1).
Small-Molecule Transmitter | Neuropeptide |
---|---|
Glutamate | Substance P |
GABA | Cholecystokinin, enkephalin, somatostatin, substance P, thyrotropin-releasing hormone |
Glycine | Neurotensin |
Acetylcholine | Calcitonin gene-related protein, enkephalin, galanin, gonadotropin-releasing hormone, neurotensin, somatostatin, substance P, vasoactive intestinal polypeptide |
Dopamine | Cholecystokinin, enkephalin, neurotensin |
Norepinephrine | Enkephalin, neuropeptide Y, neurotensin, somatostatin, vasopressin |
Epinephrine | Enkephalin, neuropeptide Y, neurotensin, substance P |
Serotonin | Cholecystokinin, enkephalin, neuropeptide Y, substance P, vasoactive intestinal polypeptide |
Figure 7–1 shows the biosynthesis of some common small-molecule transmitters released by neurons in the central nervous system (CNS) or peripheral nervous system. Figure 7–2 shows the location of major groups of neurons that contain norepinephrine, epinephrine, dopamine, and acetylcholine. These are some of the major central neurotransmitter and neuromodulatory systems.
FIGURE 7–1
Biosynthesis of some common small-molecule neurotransmitters. A) Glutamate is synthesized in the Krebs cycle by the conversion of α-ketoglutarate to the amino acid via the enzyme γ-aminobutyric acid transferase (GABA-T) or in nerve terminals by the hydrolysis of glutamine by the enzyme glutaminase. GABA is synthesized by the conversion of glutamate by the enzyme glutamic acid decarboxylase (GAD). B) Acetylcholine is synthesized in the cytoplasm of a nerve terminal from acetyl-Co-A and choline by the enzyme choline acetyltransferase. C) Serotonin is synthesized from the amino acid tryptophan in a two-step process: the enzymatic hydroxylation of tryptophan to 5-hydroxytryptophan and the enzymatic decarboxylation of this intermediate to form 5-hydroxytryptamine (also called serotonin). D) Catecholamines are synthesized from the amino acid tyrosine by a multi-step process. Tyrosine is oxidized to dihydroxyphenylalanine (DOPA) by the enzyme tyrosine hydroxylase in the cytoplasm of the neuron; DOPA is then decarboxylated to dopamine. In dopaminergic neurons, the process stops there. In noradrenergic neurons, the dopamine is transported into synaptic vesicles where it is converted to norepinephrine by dopamine-β-hydroxylase. In neurons that also contain the enzyme phenylethanolamine-N-methyltransferase, norepinephrine is converted to epinephrine.
FIGURE 7–2
Four diffusely connected systems of central neuromodulators. A) Noradrenergic neurons in the locus coeruleus innervate the spinal cord, cerebellum, several nuclei of the hypothalamus, thalamus, basal telencephalon, and neocortex. B) Serotonergic neurons in the raphe nuclei project to the hypothalamus, limbic system, neocortex, cerebellum, and spinal cord. C) Dopaminergic neurons in the substantia nigra project to the striatum and those in the ventral tegmental area of the midbrain project to the prefrontal cortex of the limbic system. D) Cholinergic neurons in the basal forebrain complex project to the hippocampus and the neocortex and those in the pontomesencephalotegmental cholinergic complex project to the dorsal thalamus and the forebrain. (Reproduced with permission from Boron WF, Boulpaep EL: Medical Physiology. St. Louis, MO: Elsevier; 2005.)
The action of a chemical mediator on its target structure is more dependent on the type of receptor on which it acts than on the properties of the mediator per se. Cloning and other molecular biology techniques have permitted spectacular advances in knowledge about the structure and function of receptors for neurotransmitters and other chemical messengers. The individual receptors, along with their ligands (the molecules that bind to them), are discussed in the following parts of this chapter. However, five themes have emerged that should be mentioned in this introductory discussion.
First, in every instance studied in detail to date, each chemical mediator has the potential to act on many subtypes of receptors. Thus, for example, norepinephrine acts on α1-, α2-, β1-, β2-, and β3-adrenergic receptors. Obviously, this multiplies the possible effects of a given ligand and makes its effects in a given cell more selective.
Second, there are receptors on the presynaptic as well as the postsynaptic elements for many secreted transmitters. One type of presynaptic receptor called an autoreceptor often inhibits further secretion of the transmitter, providing feedback control. For example, norepinephrine acts on α2-presynaptic receptors to inhibit additional norepinephrine secretion. A second type of presynaptic receptor is called a heteroreceptor whose ligand is a chemical other than the transmitter released by the nerve ending on which the receptor is located. For example, norepinephrine acts on a heteroreceptor on a cholinergic nerve terminal to inhibit the release of acetylcholine. In some cases, presynaptic receptors facilitate the release of neurotransmitters.
Third, although there are many neurotransmitters and many subtypes of receptors for each ligand, the receptors tend to group in two large families in terms of structure and function: ligand-gated channels (also known as ionotropic receptors) and metabotropic receptors. In the case of ionotropic receptors, a membrane channel is opened when a ligand binds to the receptor; and activation of the channel usually elicits a brief (few to tens of milliseconds) increase in ionic conductance. Thus, these receptors are important for fast synaptic transmission. Metabotropic receptors are 7-transmembrane G-protein–coupled receptors (GPCR), and binding of a neurotransmitter to these receptors initiates the production of a second messenger that modulates the voltage-gated channels on neuronal membranes. The receptors for some neurotransmitters and neuromodulators are listed in Table 7–2, along with their principal second messengers and, where established, their net effect on ion channels. It should be noted that this table is an over-simplification. For example, activation of α2-adrenergic receptors decreases intracellular cyclic adenosine monophosphate (cAMP) concentrations, but there is evidence that the G-protein activated by α2-adrenergic presynaptic receptors also acts directly on Ca2+ channels to inhibit norepinephrine release.
Neurotransmitter | Receptor | Second Messenger | Net Channel Effects | Agonists | Antagonists |
---|---|---|---|---|---|
Glutamate | AMPA | ↑Na+, K+ | AMPA | CNQX, DNQX | |
Kainate | ↑Na+, K+ | Kainate | CNQX, DNQX | ||
NMDA | ↑Na+, K+, Ca2+ | NMDA | AP5, AP7 | ||
mGluR1 | ↑cAMP, IP3, DAG | ↓K+,, ↑Ca2+ | DHPG | ||
mGluR5 | ↑IP3, DAG | ↓K+,, ↓Ca2+ | Quisqualate | ||
mGluR2, mGluR3 | ↓cAMP | ↓K+, Ca2+ | DCG-IV | ||
mGluR4, mGluR6-7 | ↓cAMP | ↓Ca2+ | L-AP4 | ||
GABA | GABAA | ↑Cl– | Muscimol | Bicuculline, Gabazine, Picrotoxin | |
GABAB | ↑IP3, DAG | ↑K+, ↓Ca2+ | Baclofen | Saclofen | |
Glycine | Glycine | ↑Cl– | Taurine, β-alanine | Strychnine | |
Acetylcholine | NM | ↑Na+, K+ | Nicotine | Tubocurarine, Gallamine triethiodide | |
NN | ↑Na+, K+ | Nicotine, lobeline | Trimethaphan | ||
M1, M3, M5 | ↑IP3, DAG | ↑Ca2+ | Muscarine, Bethanechol, Oxotremorine (M1) | Atropine, Pirenzipine (M1) | |
M2, M4 | ↓cAMP | ↑K+ | Muscarine, Bethanechol (M2) | Atropine, Tropicamide (M4) | |
Norepinephrine | α1 | ↑IP3, DAG | ↓K+ | Phenylephrine | Prazosin, Tamsulosin |
α2 | ↓cAMP | ↑K+, ↓Ca2+ | Clonidine | Yohimbine | |
β1 | ↑cAMP | ↓K+ | Isoproterenol, Dobutamine | Atenolol, Esmolol | |
β2 | ↑cAMP | Albuterol | Butoxamine | ||
Serotonin | 5HT1A | ↓cAMP | ↑K+ | 8-OH-DPAT | Metergoline, Spiperone |
5HT1B | ↓cAMP | Sumatriptan | |||
5HT1D | ↓cAMP | ↓K+ | Sumatriptan | ||
5HT2A | ↑IP3, DAG | ↓K+ | Dobutamine | Ketanserin | |
5HT2C | ↑IP3, DAG | α-Methyl-5-HT | |||
5HT3 | ↑Na+ | α-Methyl-5-HT | Ondansetron | ||
5HT4 | ↑cAMP | ↓K+ | 5-Methoxytryptamine |
Fourth, receptors are concentrated in clusters on the postsynaptic membrane close to the endings of neurons that secrete the neurotransmitters specific for them. This is generally due to the presence of specific binding proteins for them.
Fifth, in response to prolonged exposure to their ligands, most receptors become unresponsive; that is, they undergo desensitization. This can be of two types: homologous desensitization, with loss of responsiveness only to the particular ligand and maintained responsiveness of the cell to other ligands; and heterologous desensitization, in which the cell becomes unresponsive to other ligands as well.
Neurotransmitters are rapidly transported from the synaptic cleft back into the cytoplasm of the neurons that secreted them via a process called reuptake, which involves a high-affinity, Na+-dependent membrane transporter. Figure 7–3 illustrates the principle of reuptake of norepinephrine released from a sympathetic postganglionic nerve. After release of norepinephrine into the synaptic cleft, it is rapidly routed back into the sympathetic nerve terminal by a norepinephrine transporter (NET). A portion of the norepinephrine that re-enters the neuron is sequestered into the synaptic vesicles through the vesicular monoamine transporter (VMAT). There are analogous membrane and vesicular transporters for other small-molecule neurotransmitters released at other synapses in the CNS and peripheral nervous system.
FIGURE 7–3
Fate of monoamines secreted at synaptic junctions. In each monoamine-secreting neuron, the monoamine is synthesized in the cytoplasm and the secretory granules and its concentration in secretory granules is maintained by the two vesicular monoamine transporters (VMAT). The monoamine is secreted by exocytosis of the granules, and it acts on G-protein–coupled receptors. In this example, the monoamine is norepinephrine acting on adrenoceptors. Many of these receptors are postsynaptic, but some are presynaptic and some are located on glia. In addition, there is extensive reuptake of the monoamine into the cytoplasm of the presynaptic terminal via a monoamine transporter, in this case the norepinephrine transporter (NET). (Modified with permission from Katzung BG, Masters SB, Trevor AJ: Basic and Clinical Pharmacology, 11th ed. New York, NY: McGraw-Hill; 2009.)
Reuptake is a major factor in terminating the action of transmitters, and when it is inhibited, the effects of transmitter release are increased and prolonged. This has clinical consequences. For example, several effective antidepressant drugs are inhibitors of the reuptake of amine transmitters, and cocaine may inhibit dopamine reuptake. Glutamate uptake into neurons and glia is important because glutamate is an excitotoxin that can kill cells by overstimulating them (see Clinical Box 7–1). There is evidence that during ischemia and anoxia, loss of neurons is increased because glutamate reuptake is inhibited.
SMALL-MOLECULE TRANSMITTERS
Synaptic physiology is a rapidly expanding, complex field that cannot be covered in detail in this book. However, it is appropriate to summarize information about the principal neurotransmitters and their receptors.
Glutamate is the main excitatory transmitter in the brain and spinal cord and has been calculated to be responsible for 75% of the excitatory transmission in the CNS. There are two distinct pathways involved in the synthesis of glutamate (Figure 7–1). In one pathway, α-ketoglutarate produced by the Krebs cycle is converted to glutamate by the enzyme GABA transaminase (GABA-T). In the second pathway, glutamate is released from the nerve terminal into the synaptic cleft by Ca2+-dependent exocytosis and transported via a glutamate reuptake transporter into glia, where it is converted to glutamine by the enzyme glutamine synthetase (Figure 7–4). Glutamine then diffuses back into the nerve terminal where it is hydrolyzed back to glutamate by the enzyme glutaminase. In addition to uptake of released glutamate into glia, the membrane transporters also return glutamate directly into the nerve terminal. Within glutamatergic neurons, glutamate is highly concentrated in synaptic vesicles by a vesicular glutamate transporter.
FIGURE 7–4
Biochemical events at a glutamatergic synapse. Glutamate (Glu) released into the synaptic cleft by Ca2+-dependent exocytosis. Released Glu can act on ionotropic and G-protein–coupled receptors on the postsynaptic neuron. Synaptic transmission is terminated by the active transport of Glu via by a Na+-dependent glutamate transporters located on membranes of the presynaptic terminal [Gt(n)] and glia [Gt(g)]. In glia, Glu is converted to glutamine (Gln) by the enzyme glutamine synthetase; Gln then diffuses into the nerve terminal where it is hydrolyzed back to Glu by the enzyme glutaminase. In the nerve terminal, Glu is highly concentrated in synaptic vesicles by a vesicular glutamate transporter.
Glutamate acts on both ionotropic and metabotropic receptors in the CNS (Figure 7–4). There are three subtypes of ionotropic glutamate receptors, each named for its relatively specific agonist. These are the AMPA (α-amino-3-hydroxy-5-methylisoxazole-4-propionate), kainate (kainate is an acid isolated from seaweed), and NMDA (N-methyl-D-aspartate) receptors. Table 7–2 summarizes some of the major properties of these receptors. Ionotropic glutamate receptors are tetramers composed of different subunits whose helical domains span the membranes three times and a short sequence that forms the channel pore. Four AMPA (GluR1–GluR4), five kainate (GluR5–GluR7, KA1, KA2), and six NMDA (NR1, NR2A–NR2D) subunits have been identified, and each is coded by a different gene.
The release of glutamate and its binding to AMPA or kainate receptors primarily permits the influx of Na+ and the efflux of K+, accounting for fast excitatory postsynaptic potential (EPSP). Most AMPA receptors have low Ca2+ permeability, but the absence of certain subunits in the receptor complex at some sites allows for the influx of Ca2+, which may contribute to the excitotoxic effect of glutamate (see Clinical Box 7–1).
Activation of the NMDA receptor permits the influx of relatively large amounts of Ca2+ along with Na+. When glutamate is in excess in the synaptic cleft, the NMDA receptor-induced influx of Ca2+ into neurons is the major basis for the excitotoxic actions of glutamate. The NMDA receptor is unique in several ways (Figure 7–5). First, glycine facilitates its function by binding to the receptor. In fact, glycine binding is essential for the receptor to respond to glutamate. Second, when glutamate binds to the NMDA receptor, it opens, but at normal membrane potentials, the channel is blocked by extracellular Mg2+. This block is removed only when the neuron containing the receptor is partially depolarized by the activation of adjacent AMPA and kainate receptors. Third, the excitatory postsynaptic potential induced by activation of NMDA receptors is slower than that elicited by activation of the AMPA and kainate receptors.
FIGURE 7–5
Diagrammatic representation of the NMDA receptor. When glycine and glutamate bind to the receptor, the closed ion channel (left) opens, but at the resting membrane potential, the channel is blocked by Mg2+ (right). This block is removed if partial depolarization is produced by other inputs to the neuron containing the receptor, and Ca2+ and Na+ enter the neuron. Blockade can also be produced by the drug dizocilpine maleate (MK-801).
CLINICAL BOX 7–1 Excitotoxins
Glutamate is usually cleared from the brain’s extracellular fluid by Na+-dependent uptake systems in neurons and glia, keeping only micromolar levels of the chemical in the extracellular fluid despite millimolar levels inside neurons. However, excessive levels of glutamate occur in response to ischemia, anoxia, hypoglycemia, or trauma. Glutamate and some of its synthetic agonists are unique in that when they act on neuronal cell bodies, they can produce so much Ca2+ influx that the neurons die. This is the reason why microinjection of these excitotoxins is used in research to produce discrete lesions that destroy neuronal cell bodies without affecting neighboring axons. Evidence is accumulating that excitotoxins play a significant role in the damage done to the brain by a stroke. When a cerebral artery is occluded, the cells in the severely ischemic area die. The surrounding partially ischemic cells may survive but lose their ability to maintain the transmembrane Na+ gradient. The elevated levels of intracellular Na+ prevent the ability of astrocytes to remove glutamate from the brain’s extracellular fluid. Therefore, glutamate accumulates to the point that excitotoxic damage and cell death occurs in the penumbra, the region around the completely infarcted area. In addition, excessive glutamate receptor activation may contribute to the pathophysiology of some neurodegenerative diseases such as amyotrophic lateral sclerosis (ALS), Parkinson disease, and Alzheimer disease.
THERAPEUTIC HIGHLIGHTSRiluzole is a voltage-gated channel blocker that may antagonize NMDA receptors. It has been shown to slow the progression of impairment and modestly improve the life expectancy of patients with ALS. Another NMDA receptor antagonist memantine has been used to slow the progressive decline in patients with Alzheimer disease. A third NMDA receptor antagonist, amantadine, in conjunction with levodopa, has been shown to improve function in patients with Parkinson disease.
Essentially all neurons in the CNS have both AMPA and NMDA receptors. Kainate receptors are located presynaptically on GABA-secreting nerve endings and postsynaptically at various sites, most notably in the hippocampus, cerebellum, and spinal cord. Kainate and AMPA receptors are found in glia as well as neurons, but NMDA receptors occur only in neurons. The concentration of NMDA receptors in the hippocampus is high, and blockade of these receptors prevents long-term potentiation, a long-lasting facilitation of transmission in neural pathways following a brief period of high-frequency stimulation. Thus, these receptors may well be involved in memory and learning (see Chapter 15).
Activation of the metabotropic glutamate receptors (mGluR) leads to either an increase in intracellular inositol 1,4,5-triphosphate (IP3) and diacylglycerol (DAG) levels or a decrease in intracellular cAMP levels (Table 7–2). There are eight known subtypes of mGluR. These receptors are located at both presynaptic (mGluR2-4, 6-8) and postsynaptic sites (mGluR1, 5
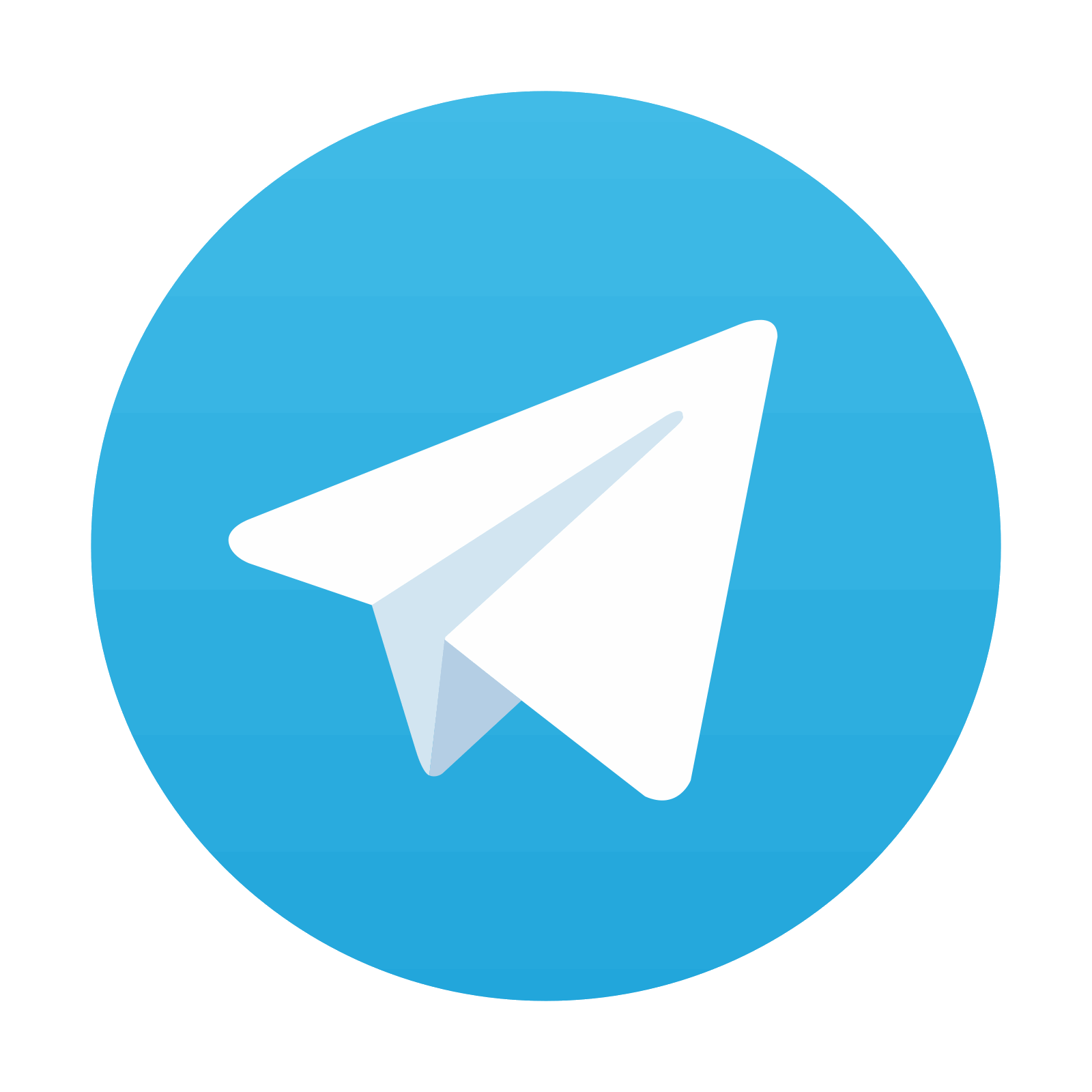
Stay updated, free articles. Join our Telegram channel
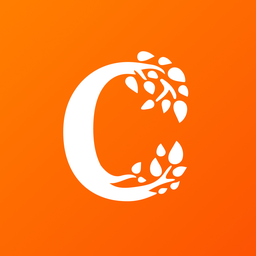
Full access? Get Clinical Tree
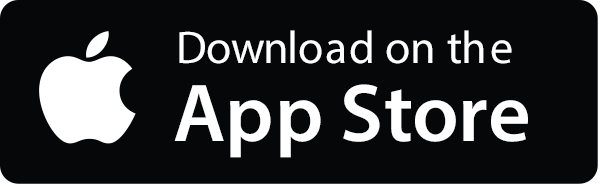
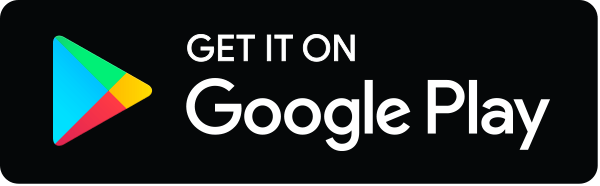
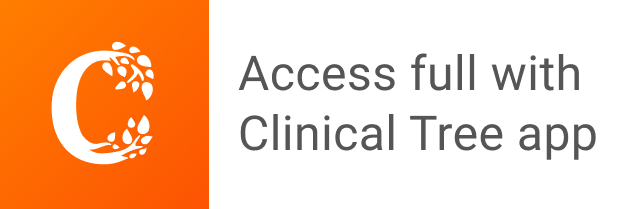