OBJECTIVES
Cranial and Cerebrovascular Disease
1 Describe the basic anatomic organization of the central nervous system in terms of hierarchical control and functional segregation.
2 Develop a common vocabulary to describe accurately the clinical findings and sites of localization to consultants and colleagues.
3 Describe the basic anatomic organization of the blood supply to the brain and the cerebrospinal fluid circulation.
4 Apply the Glasgow Coma Scale to assessment of level of consciousness.
5 Describe the clinical findings that lead one to suspect each of the herniation syndromes.
6 List the steps in the determination of brain death, and explain their significance.
7 List and explain the indications for and contraindications to lumbar puncture.
8 Discuss the treatment alternatives and sequential steps in medically stabilizing elevated intracranial pressure, the risks associated with hyperventilation, as well as the indications for, and different types of, intracranial pressure monitors.
9 Describe the initial evaluation of a comatose patient with a head injury.
10 List the different types of intracranial lesions seen on early neuroimaging for closed head injury; discuss their significance for patient outcome and their potential for surgical reversal.
11 List the features of a basilar skull fracture that would raise one’s suspicion for a traumatic vascular injury to the carotid artery.
12 Describe the typical setting and clinical and neuroimaging findings associated with pediatric shakenwhiplash syndrome and understand the physician’s responsibility in reporting this syndrome for child abuse investigation.
13 Describe the management of scalp lacerations and penetrating injuries.
14 Define transient ischemic attack, list the most common sources, and describe its relationship to cerebral infarction.
15 List the clinical and radiologic features that differentiate ischemic stroke from hemorrhagic stroke. List the disorders that can produce each type.
16 Describe the role and timing of carotid endarterectomy and endovascular thrombolysis in patients with cerebrovascular disease.
17 Describe the danger associated with aneurysmal subarachnoid hemorrhage, define and discuss the frequency of sentinel bleeds, and describe the relative roles of CT scanning and lumbar puncture in diagnosing subarachnoid hemorrhage
18 List the common brain tumors that affect adults and children, the prognosis for each, and the symptoms that herald a brain tumor.
19 Based on the history and physical examination, choose and interpret the diagnostic tests needed to establish the site and nature of the tumor.
20 List examples of tumors and clinical circumstances that might benefit from stereotactic biopsy, open surgical excision, stereotactic radiosurgery, fractionated radiotherapy, and chemotherapy for brain tumors
21 Describe the symptoms, evaluation, and treatment of a patient with a brain abscess
22 Describe the role and alternative techniques of diagnostic brain biopsy for patients with nonbacterial central nervous system infections
23 Describe the symptoms and evaluation of a patient with suspected hydrocephalus
24 Describe the complications of ventricular shunting
25 Describe the shape of the head with premature closure of the cranial sutures: the sagittal suture, one coronal suture, both coronal sutures, and the lambdoid suture
26 Describe the signs and symptoms of Chiari I and Chiari II malformation and the goals of surgical intervention for these conditions
27 Describe the significance of a new-onset seizure in an adult and distinguish this circumstance from epilepsy.
28 Define intractability for patients with epilepsy, list at east two invasive epilepsy monitoring techniques, describe the most common surgical epilepsy procedures, and list the resulting benefits of the procedures from the standpoint of seizure control, cognitive function, employability, and quality of life
29 Describe the role of surgery in patients with Parkinson’s disease, and list the lesional and stimulator surgical options available for these patients
30 Describe the symptoms of normal pressure hydrocephalus, as well as the diagnostic procedures that correlate with improvement after ventricular shunting
Spine and Spinal Cord Disease
1 Describe the clinical findings that lead one to suspect each of the spinal cord syndromes, and compare and contrast cauda equine and conus medullaris syndrome
2 List the most common causes of each of the spina cord syndromes
3 Describe the three-column theory of for inferring spinal column stability based on anatomic disruption
4 List the important steps in the evaluation and management of acute spinal cord injury. Consider bodily injury, spinal instability, and spinal cord damage
5 Describe the diagnostic name, anatomy of the break, and findings on neuroimaging of three stable C1-2 fractures
6 Describe the diagnosis and significance of Spina Cord Injury Without Radiographic Abnormality (SCIW-ORA) in the pediatric population
7 Describe the initial imaging findings, initial stabilization methods, early reduction steps, and indications for surgery for subaxial C3-C7 fractures
8 Describe the difference between a compression and burst fracture, and list the indications for surgical stabilization
9 Define dermatome and myotome, describe the symptoms and findings of radiculopathy, and list the most common causes of radiculopathy.
10 Describe the signs and symptoms of neurogenic claudication and describe its relationship to lumbar stenosis
11 Describe the clinical manifestations, diagnostic workup, conservative management, and surgical indications for a patient with cervical disc herniation and umbar disc herniation
12 Describe the clinical manifestations, diagnostic workup, conservative management, surgical indication, and different surgical approaches for a patient with degenerative cervical stenosis and lumbar stenosis.
13 List the pathologic features, clinical symptoms and signs, and surgical corrective measures for spondylolisthesis
14 Describe the vascular supply to the anterior and posterior spinal cord, as well as the differential supply to the cervical and upper thoracic versus lower thoracic and lumbar-sacral spinal cord
15 Describe the differential clinical signs and symptoms that distinguish anterior spinal artery spinal cord infarction from compression by epidural hematoma
16 Describe the common route of spread for metastatic carcinoma and the corresponding findings on neuroimaging, and compare this with the common route of spread to the spine and imaging findings for lymphoma.
17 Describe the relative and appropriate roles for steroid treatment, emergent fractionated radiotherapy, surgical decompression, and surgical fusion for metatstatic cord compression
18 Describe the single most important prognostic factor for determining whether a patient will have functionally independent ambulatory function after surgical decompression for metastatic spinal cord compression
19 List the common intradural intramedullary and extra-medulalry spinal cord tumors, and compare their typical modes of presentation
20 List the social and medical risk factors for spontaneous discitis, and describe the typical radiographic findings for this condition
21 Describe the differences seen on neuroimaging between bacterial discitis and osteomyelitis and tuberculous spine disease
22 Describe the medical and surgical treatments for common spine infections
23 Differentiate between meningocele and myelomeningocele according to deficits and appearance. Describe and compare them with respect to associated neural and other problems
24 List at least two other central nervous system conditions associated with spina bifida, and describe the significance of folate for changing the incidence of this developmental anomaly.
25 Describe the relative growth features of musculoskeletal somites and central nervous system spinal cord segmental innervation during development as it relates to the formation of the cauda equine and its significance for tethered cord syndrome
Peripheral Nerve Disease
1 Describe the cross-sectional anatomy of a periphera nerve
2 Describe the role of the brachial and lumbar-sacral plexus in mixing dermatomes and myotomes into peripheral nerve distributions
3 Define neuropraxia, incomplete nerve transection, and complete nerve transection. Describe the means to differentiate them
4 Compare the healing of a crushed nerve, a transected nerve, and a surgically joined nerve
5 List the factors that affect the timing of periphera nerve repair.
6 Describe the role of electromyography in distinguishing between radiculopathy and peripheral neuropathy, defining the level of traumatic peripheral nerve or plexus injury, as well as the course of recovery after injury or surgical repair
7 Describe the features that distinguish carpal tunnel syndrome, tardy ulnar palsy, and cervical disc disease
8 List the treatable medical risk factors for potentially medically reversible nerve entrapment neuropathies
9 Describe the role of electromyography and nerve conduction studies in confirming and localizing pathologic nerve entrapment.
10 Describe the relationship of neurofibromatosis to schwannomas and neurofibromas and the worrisome signs and symptoms for malignant degeneration in patients with neurofibromas
Pain
1 Describe the characteristic signs and symptoms, typical natural history, as well as early medical treatment of trigeminal neuralgia and glossopharyngea neuralgia
2 Describe the potentially curative as well as palliative surgical options available for treating patients with trigeminal neuralgia, as well as the general efficacy and recurrence rates for each
3 Describe the surgical options available for treating patients with glossopharyngeal neuralgia
4 Describe the gating control theory of pain
5 Describe the potential downside of peripheral deafferentation procedures for pain syndromes
6 Describe the surgical options for treating painful neuromas
7 Describe at least two chronic pain syndromes that may respond to sympathectomy.
8 List and describe surgically implantable pharmacologic and stimulatory spinal cord strategies for treating chronic neuropathic and terminal cancer pain syndromes
9 List and describe stereotactic lesioning and stimulatory deep brain strategies for treating chronic neuropathic and terminal cancer pain syndromes
10 List at least three chronic pain syndromes that have shown therapeutic response to motor cortex stimulation
Neurological surgery is a relatively young specialty field that evolved as a separate discipline from general surgery during the early 1900s. While often referred to as “brain surgery,” modern practice management surveys reveal that general community neurosurgical practice comprises approximately 75% to 80% spinal surgery, 20% cranial and cerebrovascular surgery, and 5% to 10% peripheral nerve surgery. Academic and university practices average roughly 50% to 60% spinal surgery, 35% to 45% cranial and cerebrovascular surgery, and 5% peripheral nerve surgery. This chapter will present an overview of neurosurgical diseases and interventions that includes cranial and cerebrovascular, spinal, peripheral nerve, pain, adult and pediatric, and congenital and acquired conditions.
CRANIAL AND CEREBROVASCULAR DISEASE
Anatomy and Physiology
Unlike other organs such as liver, lungs, kidneys, and muscles, in which cells are organized into repetitive identical units (e.g., lobules, alveoli, glomeruli), the central nervous system (CNS) is heterogeneous and is hierarchically organized at many different levels. Involuntary motive force for activities such as consciousness, breathing, blood pressure, and heartbeat have intrinsic pacemakers within the brain stem, which is divided into the midbrain, pons, and medulla (see Figs. 8-1 and 8-2; shown in Fig. 8-12). Voluntary activities including thinking, communicating, motor movements, and behavior have their origin in the lobes of the cerebral hemispheres (frontal, parietal, temporal, and occipital), which send motor efferent and receive sensory afferent signals down through the brain stem and the spinal cord to reach the rest of the body via the cranial and peripheral nerves, as well as the autonomic nervous system. These afferent and efferent connections are modulated and modified by superimposed secondary circuitry from the cerebellum, striatum, subthalamus, and red nucleus. The thalamus serves as a relay station and connection module for afferent primary and all secondary circuitry. Through its control of the pituitary gland, the hypothalamus serves as the source of autonomic tone and impulses, the modulator of body homeostasis (temperature, serum osmolarity), and the master regulator of hormonal levels and temporal rhythms. Knowledge of CNS functional organization and regional specialization, along with a thorough neurologic examination, are the keys to determining the level and location of a CNS lesion.
Figure 8-1 Medial aspect of the left side of the brain. The brain stem is displaced caudally for purposes of illustration.
Figure 8-2 Lateral view of the left side of the brain. The brain stem is displaced caudally for purposes of illustration.
The intracranial cavity is divided into two chambers by a fibrous curtain called the tentorium. The tentorium has an opening (tentorial incisura) through which the brain stem travels to connect with the cerebral hemispheres. Both cerebral hemispheres occupy the supratentorial space. The supratentorial space is divided into two lateral compartments by an incomplete curtain of dura called the falx cerebri, which extends the length of the interhemispheric fissure. The hemispheres are composed of an outer cortical layer (gray matter—containing neurons), a middle layer of white matter (containing axons), and an inner mass of gray matter (diencephalon, thalamus, and hypothalamus—containing neurons) (Figs. 8-1 to 8-3). The outer cortex is folded into ridges (gyri) separated by fissures (sulci). The frontal lobe, which occupies the anterior cranial fossa, is separated from the parietal lobe by the Rolandic fissure (i.e., central sulcus—see Fig. 8-1) and from the temporal lobe, which sits in the temporal bone and the tentorium, by the sylvian fissure (Fig. 8-1). The occipital lobe occupies the posterior pole of each hemisphere. The frontal lobe is the primary source of motor function. Lesions in the posterior portion cause contralateral weakness and hyperreflexia. The primary motor strip lies just in front, and the primary sensory strip just behind, the central sulcus. Lesions of the anterior parietal lobe cause contralateral sensory dysfunction. The visual pathways course from the optic nerves through optic chiasm and tracts to the lateral geniculate body of the thalamus. From there, the white matter connections course through the posterior temporal lobe (Meyer’s loop) and through the parietal lobe to finally end on the primary calcarine cortex in the interhemispheric surface of the occipital lobe. Lesions of the temporal lobe visual pathways lead to a contralateral superior visual field defect (superior quadrantanopsia), lesions of the parietal visual connections cause an inferior visual defect (inferior quadrantanopsia), and lesions of the primary visual cortex cause a contralateral complete visual field defect (hemianopsia).
Figure 8-3 Coronal section of the brain showing the gray matter (stippled).
Although primary motor and sensory functions are symmetrically organized bilaterally, some higher cortical functions are represented only in one hemisphere or the other. Speech dominance is an example. Ninety-five percent of naturally right-handed people have speech located in the left hemisphere, while 50% of naturally left-handed people have speech located in the right hemisphere. For left brain–dominant people, lesions of the inferior-lateral left frontal lobe, just above the root of the sylvian fissure (Broca’s area), cause an expressive (nonfluent) aphasia. Lesions in the posterior-superior temporal lobe, just below the end of the sylvian fissure (Wernicke’s area) cause loss of speech comprehension and a fluent aphasia consisting of meaningless sounds or words. Lesions of the connection between the two areas lead to a conductive aphasia, in which speech is understood but the patient is unable to repeat the understood phrase. Other examples include calculations, which localize to the right parietal lobe, and pattern recognition and spatial orientation, which localize to the right parietal lobe in left brain–dominant people
Some functions do not respect lobar boundaries, but involve connections throughout all four. An example is the limbic system, which consists of concentrically paired looping circuits involving the medial temporal lobe (amygdala, hippocampus, and parahippocampal gyrus), the medial parietal lobe (posterior cingulated gyrus), the inferior medial occipital lobe (posterior occipital-temporal gyrus), the medial and inferior frontal lobe (anterior cingulated gyrus and the medial septal nuclei), the fornix, and the hypothalamus. The limbic system is the entry portal for short-term memory and is intimately involved in learning, as well as regulating mood and affect. However, only bilateral lesions are usually symptomatic. Still other functions such as long-term memory are diffusely stored in ways we have yet to fully understand.
The cortical functions of the four lobes within one hemisphere connect with one another via subcortical white matter tracts oriented anteriorly-posteriorly, including the superior and inferior longitudinal fasciculi. They connect one hemisphere to the other by way of laterally oriented white matter tracts across the corpus callosum, as well as the anterior and posterior commissures. They connect with the brain stem and spinal cord by way of a condensation of white matter tracts called the internal capsule (Fig. 8-3; shown in Fig. 8-9E), which are oriented in a rostral-caudal direction. The internal capsule passes through the diencephalon between the basal ganglia. The caudate and putamen (striatum) lie laterally and the thalamus medially to the internal capsule (Fig. 8-3; shown in Figs. 8-9E and 8-10). Even very small vascular lesions in this tight condensation of white matter connections can lead to severe weakness (lacunar strokes). The nonspecific nuclei of the thalamus are extensions of the reticular formation of the brain stem and are involved with arousal and alertness. When they are damaged (e.g., thalamic hemorrhage), coma occurs. The thalamus acts as a relay point for sensation. Somatosensory input from the spinal cord and brain stem is processed in the ventral posterior thalamus and relayed to the parietal lobe. Special sensory afferent information is relayed to the cortex from the posterior thalamus (hearing from the medial geniculate nucleus to the temporal lobe and vision from the lateral geniculate nucleus to the occipital lobe). Input from the limbic system related to memory and emotion is also connected through the thalamus. The anterior nuclei receive limbic input from the mamillary bodies of the hypothalamus (to which the hippocampus projects) and project to the medial cortex of the frontal lobe (cingulate gyrus).
The infratentorial compartment of the cranium (the posterior fossa) contains the brain stem and the cerebellum. The brain stem connects inferiorly with the spinal cord byway of an opening in the skull called the foramen magnum. The cerebellum lies on the back of the brain stem like a papoose and connects with the brain stem by way of three axially oriented white matter tracts on each side (the superior, middle, and inferior cerebellar peduncles, respectively—Fig. 8-1; shown in Fig. 8-12). The cerebellum is involved with modulation and coordination of motor movements by means of inhibitory modulatory inputs. The paired lateral hemispheres control the limbs, and lesions here lead to dysmetria. The central vermis controls the axial musculature, and lesions here cause ataxia. The inferior tonsils as well as the inferior-lateral flocculus and nodulus control vestibular-ocular coordination, and lesions here cause nystagmus.
The brain stem is divided into a dorsal component (tectum) that contains the reticular formation, cranial nerve nuclei, and lemniscal sensory tracts. The ventral component (tegmentum) contains the motor white matter connections, the cerebellar modulatory connections, and the cranial nerve roots. The motor pyramidal tract is densely compacted and lies very close to the ventral surface of the brain stem at two locations. In each of the locations it is susceptible to dysfunction form external pressure. The first is in the midbrain cerebral peduncle, where contralateral hemiparesis occurs with compression by the medial hippocampus during transtentorial herniation. The second is the decussation of the pyramids in the ventral medulla, where ventral skull-base tumors can cause confusing motor weakness patterns (cruciate paralysis). Most of the long white matter tracts that pass between the spinal cord and the brain stem cross from side-to-side at the spinal medullary junction. The level of lesions in the brain stem can be determined from the level of cranial nerve involvement. Cranial nerves 3 and 4 arise from the midbrain. Cranial nerves 5, 6, 7, and 8 come from the pons. Cranial nerves 9, 10, 11, and 12 arise from the medulla (Fig. 8-2). All cranial nerves exit the brain stem ventral-laterally except for the fourth cranial nerve, which exits dorsally. The reticular formation of the brain stem controls respiration, heart rate, blood pressure, and consciousness.
Blood Supply
The brain receives 20% of the stroke volume of each heartbeat. Four major blood vessels course through the neck from the apical chest vessels to supply the brain. The two common carotid arteries divide at the carotid bifurcation into the internal carotid artery (ICA) and external carotid artery (ECA). The ECA supplies blood to the face, the scalp, and the meningeal covering of the brain by way of several terminal arteries, including the middle meningeal artery (MMA). The major ECA scalp terminal branches are the superficial temporal artery (STA) coursing just in front of the ear and the occipital artery coursing just behind the ear. The ICA courses through the petrous canal of the skull base to the cavernous sinus located on either side of the pituitary gland. It supplies the ophthalmic branch to the orbit and then penetrates the dura covering of the brain. The carotid system supplies 80% of the blood supply to the brain, with each ICA supplying approximately 40%. The two vertebral arteries (VA) together supply 20% of the brain’s blood volume. Most people have a left-brain dominant VA, fewer have a balanced vertebral system, and a small number have a right-dominant VA. The VAs enter the transverse foramen of the cervical vertebra at C6 and segmentally course through each transverse foramen all the way up through C2. They penetrate the dura between the arch of C1 and the posterior-lateral rim of the foramen magnum. The VA is susceptible to injury (dissection or occlusion, with subsequent ischemia or embolization) with sudden spinal movements or with spine fractures involving the transverse foramen.
The posterior fossa is the only location in the human body where vessels (in this case the VAs), instead of branching as they extend further from the heart, join to form a single distal posterior circulation artery, the basilar artery (BA). Before joining to form the BA, each VA gives off a posterior inferior cerebellar artery (PICA), which supplies the lateral medulla and the inferior-lateral cerebellum. At the mid pons level, the BA gives off bilateral anterior-inferior cerebellar arteries (AICA), which supply the lateral pons and cerebellum. Near its terminus, the BA gives off bilateral superior cerebellar arteries (SCA), which supply the lateral brain stem and the superior and superior-lateral cerebellum. The BA ends in a bifurcation consisting of the initial segments of the posterior cerebral arteries (PCA), which supply the inferior temporal lobe, the occipital lobe, and the posterior-medial parietal lobe. The ICAs give off posteriorly coursing posterior communicating arteries (pCom A) on each side, which join with the PCAs at the level of the midbrain. They then continue, bifurcating into the middle cerebral artery (MCA) and the anterior cerebral arteries (ACA). The ACAs course medially under the frontal lobes and connect with each other by way of an anterior communicating artery (aCom A), before continuing up the interhemispheric fissure to supply the frontal pole and the medial frontal and medial anterior parietal lobes. The MCA courses up the sylvian fissure to supply the whole lateral portion of the frontal, temporal, and parietal lobes. Because it carries the largest volume of blood flow and supplies the largest volume of brain, the MCA distribution is the most common distribution to receive a vascular embolus (embolic stroke or metastatic brain tumor). Normal, average, mixed cortical cerebral blood flow (CBF) to the brain is 55 mL/100 g/min (SD ± 12); white matter values are approximately 22 mL/100 g/min.
The base of the brain in the arachnoid cisterns contains a unique collateral supply system called the circle of Willis. It consists of the ICA, the first segments of both ACAs, the aCom A, both pCom As, and the first segments of both PCAs. These segments connect in a geometric pentagon, with the short aCom A segment constituting the blunted apex. Through the circle of Willis any one ICA and one VA could theoretically supply blood to any area of the brain. In reality, the circle of Willis is quite variable in integrity and symmetry, and even angiographic demonstration of an intact ring does not guarantee adequate collateral potential. Only 75% to 80% of people will tolerate the sudden loss or occlusion of one ICA without stroke. Five percent will become symptomatic within 5 to 15 minutes of occlusion from flow-related ischemia, and 15% to 20% will initially be asymptomatic, but will have marginal reserves that carry a high risk of delayed stroke with any subsequent episode of dehydration or hypotension.
Venous drainage of the brain has both deep and superficial components. Deep in the brain, the two paired thalamostriate and intracerebral veins drain the diencephalon and join the two basal veins of Rosenthal, which drain the upper brain stem to form the single, short, and deep vein of Galen. The inferior sagittal sinus, which runs along the inferior edge of the falx cerebri dural reflection separating the hemispheres, joins the vein of Galen. Here, the falx joins the tentorium to form the straight sinus. The straight sinus runs along the falx-tentorium insertion to join the torcula. Superficially, the sylvian vein (the vein of Labbé), coursing in the sylvian fissure, drains the brain. This vein runs from the top of the sylvian fissure down along the posterior-lateral aspect of the temporal lobe to join the transverse sinus. The vein of Trolard runs upward from the top of the sylvian fissure along the central sulcus to join the sagittal sinus. Together, the sylvian vein, vein of Labbe, and vein of Trolard form a crude venous Mercedes-Benz symbol on each side of the brain. Multiple smaller lateral cortical veins run upward to join the sagittal sinus by bridging across the subarachnoid space to the dura. These “bridging veins” are particularly prone to tearing with lateral shear forces. They are a major source of both acute traumatic and spontaneous chronic subdural hematomas. Ultimately, the sagittal sinus flows back to join the two transverse sinuses, along with the straight sinus at a junction called the torcula. The two transverse sinuses then flow laterally to join the sigmoid sinuses behind each ear, which then flow down to finally become the internal jugular veins bilaterally. A minor anterior venous drainage collateral system courses through the cavernous sinus and the pterygopalatine plexus of the face.
Cerebrospinal Fluid Circulation
During life, the brain has the texture and consistency of uncooked tofu. Thus, it is susceptible to deformation and shearing forces with sudden accelerations and decelerations. Fortunately, it has an internal structural skeleton in the form of fluid-filled cerebral ventricles and an external fluid cushioning layer in the form of the subarachnoid space and the basal arachnoid cisterns. Cerebrospinal fluid (CSF) performs this structural and cushioning role; it also forms a second circulation for the brain, helping to maintain and regulate cerebral and systemic homeostasis. It is produced by choroid plexus in an energy-dependent, carbonic anhydrase–dependent, active process at the rate of 0.3 mL/min (totaling 432 mL/day). Because the total volume of CSF in the cranium and spinal canal is approximately 150 mL, this means that the total volume of CSF is completely replaced 3 times per day.
The cerebral hemispheres contain paired lateral ventricles that form the internal core of all four lobes. They form a large medial-leaning “C” with a posterior extension into the occipital lobe (occipital horn). The two lateral ventricles drain into a single midline third ventricle, which lies between the two diencephalons just above the midbrain by way of the paired foramen of Monroe (Fig. 8-4). Only the anterior horn of the lateral ventricle does not contain choroid plexus. The third ventricle drains through the midbrain by way of the single narrow aqueduct of Sylvius to join the fourth ventricle, which lies in the posterior fossa between the brainstem in front and the cerebellum behind. Fluid then exits the ventricular system either by way of the single medial foramen of Magendie (located between the paired tonsils of the cerebellum), draining into the cisterna magna, or laterally via two foramina of Luschka, draining into the cerebellopontine angle on either side. The CSF flows down the spinal canal, under the brain through the basal arachnoid cisterns, and over the convexity of the brain in the subarachnoid space. It is absorbed into the venous system at the dural venous sinuses by way of arachnoid villi. These villi serve as oneway communication valves, with absorption driven by the pressure gradient between the subarachnoid space and the venous pressure in the dural sinuses.
Figure 8-4 Circulation of the cerebrospinal fluid.
Intracranial Pressure, Cerebral Perfusion Pressure, Cerebral Autoregulation, and Brain Herniation
The skull is a closed, rigid chamber with both fixed and variable content volumes. Under normal conditions, the cranial cavity contains only brain, CSF, and blood. Normal intracranial pressure (ICP) in an adult is less than 10 to 15 cm of H2O (14–20 mm Hg). In children, before skull growth-plate fusion, it is much lower. According to the Monroe-Kellie hypothesis, in a fixed, enclosed space adding additional masses to the fixed volume (e.g., blood clots, swollen contusions, tumors, large strokes, excess CSF) will lead to stable intracranial pressure only so long as the buffering capacity of increased CSF absorption can keep up. At some point, this buffering capacity is saturated, and at this point even small extra volume increases will lead to very large increases in ICP. Indeed, ICP will rise exponentially with additional volume increases from this point onward.
The brain volume is fixed in both the supratentorial and infratentorial compartments. It is also fixed in each lateral compartment of the supratentorial space (separated by the falx cerebri). The CSF volume is relatively fixed, with only small increases in CSF absorption possible by increasing the pressure gradient across the arachnoid villi–dural sinus interface. The blood volume is the most variable of the three because elevated ICP can prevent inflow of blood into the cranial cavity. It is therefore the most susceptible to pathologic derangement resulting from conditions that raise ICP, with the potential for secondary ischemic injury to the brain. Cerebral perfusion pressure (CPP) is the difference between mean systemic arterial pressure (MAP) and ICP (CPP = MAP – ICP). Normal CPP is >50 mm Hg (usually in the range of 55–65 mm Hg). In general, when CPP falls below 45 mm Hg , the brain is at risk for developing areas of reduced CBF, depending on local-regional conditions for the area of brain at risk.
Cerebral autoregulation is a vasoconstriction-dilatation compensation mechanism that normally keeps CBF constant over a wide range of blood pressures (MAP 40–140 mm Hg). In addition to the actual CBF, the arterial level of CO2 is the second main arbiter of the degree of cerebral vascular tone (vasoconstriction with low levels of CO2 and vasodilatation with high levels). Intracranial pressure can be lowered by hyperventilating the patient and lowering arterial CO2, but only at the expense of lowering CBF to a brain that may be very susceptible to secondary injury with any further ischemia. With brain injury from trauma or ischemia, autoregulation can be disrupted in local regions of the brain, and with repeated or prolonged elevations of CPP, autoregulation can fail throughout the brain. When this occurs, CBF is dependent on CPP in a linear manner.
A hernia is the physical displacement of tissue from one compartment into another due to a pressure gradient across the opening between the chambers in question. This definition and concept is the same for the inguinal canal, the diaphragm, or the cranial cavity. Because the cranium has several compartments, several types of herniation are possible, including: (a) transtentorial herniation (tissue moving downward from the supratentorial space through the tentorial incisura into the infratentorial space); (b) tonsillar or downward herniation (tissue moving from the posterior fossa through the foramen magnum); (c) subfalcine herniation (tissue moving from one side of the supratentorial space to the other under the falx cerebri); (d) upward central herniation (tissue moving upward from the infratentorial space through the tentorial incisura into the supratentorial space); and (e) herniation of tissue outside the skull through a craniotomy or traumatic skull defect. With herniation syndromes, it is the physical mass effect from the herniating tissue pressing on the tissue in the recipient compartment that causes dysfunction and damage. High ICP alone will not lead to herniation so long as the pressures are equal across compartments. Patients with pseudotumor cerebri routinely exhibit ICPs in the 20 to 30 cm H2O range without herniation because the pressures are equal across all compartments.
Evaluation
The Neurologic Examination
An organized and systematic approach to the neurologic examination is the best way to ensure thoroughness. The examination should include assessment of: (a) mental status, (b) cranial nerves, (c) cerebellar function, (d) motor and reflex function, and (e) sensory function. Initial elective evaluations require detail and depth in each portion. Subsequent follow-up examinations and emergency evaluations should be tailored to go into clinically relevant detail as necessary.
Mental status examination includes assessment of level of consciousness; orientation to person, place, and time; speech comprehension and expression (best tested with repetition of phrases); immediate and short-term memory; mental arithmetic; writing; and drawing. Descriptions should include the specifics of both stimulus attempted and the response obtained. For patients with head injury, the Glasgow Coma Scale (GCS) with scores extending from 3 to 15 is very useful and has been verified for reproducibility and interobserver variability (Table 8-1). A patient with a score of 8 or less is considered to be comatose. For nontrauma patients, a useful objective and quantifiable format is the Mini-Mental Status Examination (Table 8-2).
Olfaction is tested in conscious and cooperative patients in each nostril separately with a nonvolatile, nonirritating odor (e.g., coffee grounds, cinnamon, vanilla). Eyes are checked for pupil size, shape, and reactivity; fullness of visual fields; presence of atrophy, papilledema, or hemorrhages on funduscopic examination; ptosis of the eyelids; fullness and absence of diplopia on extraocular movements (EOMs); and the presence or absence of nystagmus. Trigeminal nerve examination includes testing facial sensation in all three trigeminal divisions, symmetry of the muscles of mastication, and presence and symmetry of corneal reflex. Facial nerve testing includes distinguishing between peripheral cranial nerve weakness (all branches) and central CNS upper motor neuron weakness (weakness limited to the mid and lower face). Hearing can be tested with a 712-Hz tuning fork for both bone conduction symmetry (midline—Weber test) and an air–bone conduction gap on either side (mastoid tip versus ear canal— Rinne’s test). In an unconscious patient, vestibular function can be assessed with the oculocephalic reflex (“doll’s eyes”) or caloric testing. In a normal state, rotating the head suddenly from side-to-side leads to the eyes maintaining a straight-ahead gaze. If they move with the rotation of the head, then they have an abnormal oculocephalic reflex. With caloric testing, cold water irrigated through a small tube in one ear canal, with the patient’s head elevated 30 degrees, depresses vestibular function of that ear, permitting unopposed function of the opposite ear and conjugate deviation of the eyes toward the irrigated ear. Absence of response is pathologic. Glossopharyngeal function is tested by both symmetry of the uvula with palate elevation and gag reflex on either side. In patients who are intubated, vagal nerve function can be tested by presence of cough reflex to deep tracheal suctioning. Shoulder shrug symmetry and tongue protrusion symmetry assess cranial nerve XI and XII function, respectively.
Cerebellar function testing includes stance and gait assessment, stability of stance with eyes closed and arms extended (Romberg’s sign), as well as appendicular assessments for dysmetria (finger-to-nose with eyes closed) and dysdiadochokinesia (finger-nose-finger with eyes open and changing target positions). The cerebellum does not function in isolation. Thus, abnormal gait can also be seen with motor hemiparesis, and nystagmus or poor balance can also indicate vestibular dysfunction.
Motor assessment in conscious patients includes analysis of symmetry of muscle bulk and tone as well as muscle strength on a scale of 0 to 5. Zero means no palpable muscle contraction, while 5 is normal strength. Three means strength enough to resist gravity but no additional resistance. Two and 4 are any assessment between 0 and 3 or between 3 and 5. In patients with altered consciousness, motor response is assessed in response to stimuli (localization, purposeful coordination, symmetry of response). A pathologic flexor response (decorticate) must be distinguished from a pathologic extensor response (decerebrate). Reflexes are rated on a scale of 0 to 4. Zero is absent reflexes, 1 is a weak reflex, and 2 is a normal reflex. Three is an overly active reflex with up to 1 to 3 beats of clonus. Four is a reflex associated with more sustained clonus. Pathologic reflexes associated with upper motor neuron lesions include extensor plantar responses (Babinski reflex), finger flexion response with sudden flexion of the distal interphalangeal joint (Hoffmann response), and ankle clonus.
Sensory examination includes both the spinothalamic pathway (pain—pin response) and the lemniscal pathway (joint position sense and symmetry of vibration sensation). Testing for pronator drift (Barrés sign — arms extended, palms up with fingers spread, with eyes closed) is a rapid way to test for both asymmetry of motor strength and integrity of joint position sense. A pathologic response includes both unilateral pronation and asymmetrically dropping one arm.
For supratentorial cerebral lesions, bilateral symmetry of examination takes on paramount importance and “lateralizing signs” (either motor, reflex, or sensory) are key findings assisting in hemispheric localization. For brain stem lesions, the level of cranial nerve involvement and the presence of decorticate or decerebrate posturing in comatose patients are critical for localizing the level of the lesion. For cerebellar lesions, lateral asymmetry of findings for hemispheric lesions and defining truncal versus appendicular findings is critical for distinguishing midline vermis versus lateral hemispheric lesions. Coma (GCS score ≤8) occurs only with diencephalic or brain stem reticular activating system dysfunction, or with global, bilateral, and diffuse cortical dysfunction.
Herniation Syndromes
Four of the five CNS herniations described above are associated with distinct clinical syndromes. Since each represents a neurologic emergency, it is very important that they be quickly recognized and addressed.
Transtentorial herniation (i.e., uncal herniation) is the most common CNS herniation syndrome. It involves the movement of the uncus and hippocampus of the medial temporal lobe medially and downward through the tentorial incisura to compress the third cranial nerve and the upper midbrain on the same side (Fig. 8-5A). The herniation results from a pressure differential between the supratentorial and infratentorial compartments and is usually associated with a lateral hemisphere mass lesion. Downward pressure on the third nerve affects the outer papillary fibers, leading to a dilated pupil on the side of the lesion (Fig. 8-5B). Pressure and torsion on the midbrain affect the reticular activating system and rapidly lead to unconsciousness. Pressure on the cerebral peduncle usually leads to contralateral hemiparesis and eventually to contralateral decorticate and then decerebrate posturing, as consciousness is lost. Rarely, pressure on the peduncle pushes the midbrain over to impact the contralateral peduncle on the edge of the tentorium without ipsilateral dysfunction (Kernohan’s notch — see Fig. 8-5C). This phenomenon occurs in <10% of transtentorial herniation syndromes, but leads to motor findings on the same side as the pupillary change. Thus change in the size of the pupil is always the most reliable lateralizing sign.
Figure 8-5 A, Extracerebral clot with local brain compression. B, Early transtentorial herniation. C, Late stage of herniation.
Central upward herniation also arises from a pressure differential between the supratentorial and infratentorial compartments. However, in this case the superior medial cerebellum moves upward through the tentorial incisura to symmetrically impact upon the upper brain stem. The result is sudden loss of consciousness from reticular activating system dysfunction and symmetrical bilateral brain stem pathologic motor findings.
Central downward herniation (i.e., tonsillar herniation) arises from a pressure differential between the infratentorial compartment and the spinal canal. The cerebellar tonsils move downward and forward to put pressure on the lower medulla. In addition to sudden loss of consciousness from reticular activating system dysfunction and bilateral brain stem extensor posturing, medullary cardiorespiratory center dysfunction leads to bradycardia and hypertension (Cushing’s reflex), as well as disrupted breathing patterns (e.g., Cheyne-Stokes respiration).
Each of the three herniation syndromes described above involves compression of the brain stem, with effects on level of consciousness through the reticular activating system. Because the brain stem is the primary involuntary respiratory and vasomotor control center for the body, all of these syndromes usually lead to death if not recognized and rapidly treated. Although the initial effects on level of consciousness can develop slowly and insidiously, each of these syndromes is characterized by rapidly accelerating clinical progression once consciousness is impaired. For this reason, consciousness and mental status remain the most important component of the neurologic examination in brain-injured patients, and medicines that affect level of consciousness (pain medications, sedatives, and other CNS depressants) must be carefully considered in these patients, unless an intracranial pressure monitor is in place.
With subfalcine herniation, the cingulated gyrus moves underneath the falx cerebri over the corpus callosum. Pressure on the ACA, which runs in this space, leads to distal ischemia in the distal distribution of that artery including the medial portion of the primary motor strip. Because this portion of the primary motor strip somatotopically maps to the leg, the patient is often symptomatic with a contralateral lower-extremity monoparesis.
Brain Death
Brain death is a legal definition of death that recognizes an irreversible physiologic state in which the brain has no function, but heartbeat and blood pressure are maintained. The determination is best made by a neurologist or neuro-surgeon. At the time of examination, there should be no evidence of significant hypothermia or potential pharmacologic cause of CNS suppression. Brain stem reflexes (pupillary, corneal, oculovestibular, caloric, gag, and respiratory) should be absent. There should be no response to trigeminal painful stimuli and no more than local motor responses (spinal) to body or limb stimulation. Disconnection of the respirator to test for the absence of spontaneous respiration is essential. This determination is carried out after a period of ventilation that allows the Pco2 to normalize. When the respirator is disconnected, adequate, steady flow of oxygen is delivered through a catheter that is introduced well down the endotracheal tube. The patient is observed for absence of spontaneous respirations. The determination is carried out until the arterial Pco2 is greater than 59 mm Hg.
Laboratory tests (electroencephalographic silence; cerebral radionuclide brain scans, cerebral angiography, or, possibly, transcranial Doppler confirmation of absent CBF) are usually considered optional, unless hypotension occurs and forces discontinuation of apnea testing. Usually, two complete examinations, which are separated in time and show no evidence of brain activity by the previously described criteria, are performed by licensed physicians before the patient is pronounced dead.
Electrolyte Disturbances of Cental Nervous System Origin
The hypothalamus is the main arbiter of systemic serum osmolality and sodium concentration, which are sensed via osmoreceptors present in areas of the hypothalamus where the blood–brain barrier is not present. The two hormones involved in this control are antidiuretic hormone (ADH) and either brain natriuretic peptide (BNP) or an upstream hormone controlling the release of BNP. Both of these hormones possibly are released from the posterior lobe of the pituitary gland (a direct extension of the hypothalamus) into the systemic blood stream. Oversecretion of either hormone leads to hyponatremia. It can precipitate herniation in a patient with a CNS mass lesion, and it can lead to seizure activity as well as make any subsequent seizure activity refractory to pharmacologic control. Loss of basal tonic ADH secretion leads to a dangerous condition known as diabetes insipidus (DI). With DI, the kidneys cannot reclaim water in the distal collecting tubules and the serum sodium and osmolarity can rise extremely fast. Sudden shifts in serum sodium (usually either up or down ≥3 mEq/L/hr) can lead to a clinically devastating demyelination syndrome of brain stem at the tegmentum-tectum junction in the pons known as central pontine myelinolysis (CPM). For reasons that are not clear, patients are most susceptible to CPM if they have concomitant liver disease (e.g., hepatitis, liver failure, liver transplant). Rapid rises in serum sodium can also lead to seizure activity, as well as make any subsequent seizure activity refractory to pharmacologic control. Thus, evaluation of serum sodium and osmolality is a very important component of the evaluation of a patient with CNS pathology.
Nonspecific brain pathology is second only to nonspecific pulmonary pathology (pneumonia, lung cancer, congestive heart failure) as the most common cause of syndrome of inappropriate ADH secretion (SIADH). SIADH can be seen in CNS conditions as diverse as meningitis, abscess, brain tumor, stroke, and intracerebral hemorrhage. The mechanism leading to hypothalamic dysfunction is poorly understood. With SIADH, the distal tubules of the kidney maximally resorb free water despite a low serum osmolarity and sodium level. The hallmark of SIADH is a high urine sodium level (>20 mEq/L). The urine sodium level indicates the severity of the SIADH episode (mild 20–99, moderate 100–150, severe >150). It is also the most sensitive indicator of syndrome resolution because it will reduce and then normalize well in advance of the serum sodium. In the absence of loop diuretics or severe kidney disease, SIADH and cerebral salt wasting are the only two conditions that will lead to abnormally high urine sodium. With SIADH the patient is hyponatremic due to hemodilution with free water and is relatively volume-overloaded, despite the common presence of intense thirst (also hypothalamically controlled). Treatment is fluid restriction for mild cases (800–1200 mL/24 hr) and/or intravenous 3% NaCl, with or without diuresis in patients in critical CNS condition or in those who have severe forms of the syndrome.
Cerebral salt wasting (CSW) is a unique syndrome that, so far, has only been described for patients with subarachnoid hemorrhage (SAH). Atrial natriuretic peptide (ANP), a hormone first described as arising from the heart, is secreted in response to atrial distention due to volume overloading. It leads to natriuresis in the kidneys. Because sodium obligates a minimum volume of water as solvent, natriuresis leads to intravascular volume reduction through natural diuresis. Recently, ANP has been found to be exactly the same as a protein derived from brain called BNP. It is not yet clear whether the hypothalamus is actually secreting the BNP by way of an atrial distention autonomic feedback loop from the heart or whether the hypothalamus can control the heart’s secretion of BNP by means of an upstream releasing factor. Either way, in patients with SAH, BNP levels can rise precipitously, leading to significant hyponatremia. The presumed mechanism of hypothalamic dysfunction is vasospasm of the perforating small arteries that supply the hypothalamus from the basal cisterns in reaction to the SAH. CSW also leads to elevated urine sodium; however, unlike patients with SIADH, patients with CSW are volume contracted. Fluid restriction in this setting is inappropriate and potentially dangerous. Treatment often requires intravenous 3% NaCl infusion. Because SIADH and CSW can occur in the same patient, often the conditions can be distinguished, and the correct measures instituted, only after inserting central venous catheters to asses patient intravascular volume status. Because CSW is seen only with SAH, many internists and medical and surgical critical care physicians are not familiar with it. It is important that neurosurgeons and neurologists carefully ensure that low serum sodium situations with high urine sodium are not all automatically treated as cases of SIADH.
The most common cause of DI is iatrogenic disruption of the hypothalamus or pituitary stalk function after surgery for hypothalamic or pituitary tumors. However, DI can also be seen in the setting of severe brain injury (trauma, intracerebral hemorrhage, major stroke, or other high ICP condition). Presumably caused by hypothalamic ischemia or infarction in these settings, it is a very dire sign that usually portends severe permanent brain damage, and often occurs as a preterminal event. Treatment of DI involves intravenous or intranasal desmopressin acetate (DDAVP), which is a short-acting ADH analog. Iatrogenic DI can be either temporary or permanent, so careful serum sodium monitoring is mandatory until the situation is more clearly defined. Patients with iatrogenic DI and relatively normal brain function can lead normal lives with administration of exogenous DDAVP, once the correct dosage and schedule is titrated.
Coagulation Disturbances of CNS Origin
The CNS has the highest concentration of tissue thromboplastin per gram of tissue homogenized of any other organ in the body. Indeed, rodent or rabbit brain was the original standard reagent utilized to trigger the prothrombin (PT) clotting test in laboratories before pure chemical reagents became available. Since tissue thromboplastin triggers the extrinsic clotting pathway, any condition in which brain tissue can become homogenized and exposed to the intra-vascular contents can lead to a consumptive systemic coagulopathy known as disseminated intravascular coagulation (DIC). With DIC, platelets, clotting factors, and fibrinogen are rapidly consumed, and fibrin degradation products rapidly rise. DIC is a life-threatening systemic coagulopathy that can be temporized by transfusions of platelets, clotting factors (fresh frozen plasma [FFP]), and fibrinogen (cryoprecipitate), but can only halt when the cause of the syndrome is removed, is cured, or exhausts itself naturally. DIC is most commonly seen in cases of severe traumatic brain injury. Rarely, it has been seen in cases of large stroke or intracerebral hemorrhage, as well as in cases of large-volume brain tumors removed through homogenation using an instrument known as a cavitronic ultrasonic aspirator. Because a large volume of tissue thromboplastin is needed to trigger systemic DIC, DIC is a marker for a major brain injury. DIC can lead to progression in a patient’s brain injury through enlargement of preexisting hematomas, conversion of contusions into hematomas, and conversion of ischemic strokes into hemorrhagic strokes. Its presence underlines the severity of the situation and usually portends a poor prognosis. Indeed, cynical students of neurology and neurosurgery often suggest that DIC in these settings stands for “death is coming.” All patients with major head injury, significant intracerebral hemorrhage, or stroke should be carefully assessed for coagulopathy.
Neuroendocrinology
The brain, through the hypothalamus, is the master controller of all hormones in the body, either through direct secretion into the systemic circulation (from posterior pituitary), or through sending releasing factors into the hypothalamic-pituitary portal circulation. Releasing factors stimulate the anterior pituitary to release its own stimulating hormones into the systemic circulation, which ultimately stimulate hormone secretion at the terminal gland (e.g., adrenal gland, thyroid, testes, ovary). It is the site that contains “thermostat” receptors, which constantly monitor the systemic levels of hormones as well as the site that integrates and coordinates hormone release with the body’s natural circadian rhythms. The previous discussion of electrolyte section covered the role of the posterior lobe of the pituitary in secreting ADH, dysfunction of which can lead to DI.
With the exception of prolactin, which is tonically suppressed by dopamine (except in women immediately postpartum, when lactation occurs), the hypothalamus and anterior pituitary gland stimulate the ultimate end-organ secretion of cortisol thyroid hormone, growth hormone, somatomedin-C (i.e., insulin-like growth factor-1 [IGF-1]), and the sex hormones (testosterone, estrogen, and progesterone). Traumatic brain injury as well as pituitary and parasellar masses can lead to varying forms and severity of hypopituitarism, which can be assessed only by measuring the hormone levels concerned. Cortisol and thyroid hormone can be particularly problematic in the neurocritical care and emergency care settings. Patients can go into Addisonian crisis because of inability to mount a stress steroid response and can have serious anesthetic consequences if they are severely hypothyroid. If there is any doubt, patients should be empirically treated intravenously with stress doses of steroids (100 mg of hydrocortisone or its equivalent every 8 hours) until their hormone status can be better defined. Pituitary tumors (adenomas) can lead to hormone hypersecretory syndromes (e.g., Cushing’s disease, acromegaly, or prolactinemia), and, in these settings, hormone testing can be diagnostic.
Lumbar Puncture
The CSF is a separate homeostatically regulated physiologic fluid unique to the CNS. Laboratory evaluation of CSF is just as important as systemic blood work in evaluating patients with CNS pathology. It is usually accessed by means of a lumbar puncture (LP) at either L4-5 or L5-S1. At these levels there is no danger of spinal cord injury, because only the cauda equina lies in the thecal sac at these levels. An LP can give a diagnostic opening pressure, which will be elevated in cases of pseudotumor cerebri, CNS infection, or high ICP from mass lesion. Standard CSF examination includes measurement of glucose level (lowered in the setting of infection), protein level (elevated in many pathologic settings), Gram stain and culture (infection), and cell count (both white cell and red cell). In the emergency room, if community acquired and potentially infectious meningitis is suspected, the CSF can be screened for antigens to the common community-acquired infectious meningitis agents. The CSF cell count assessment is particularly important. Although white cells will be elevated (>5) in the setting of many infections, a neutrophil predominance suggests bacterial process, while a mononuclear predominance suggests a viral, fungal, or noninfectious inflammatory condition. An elevated CSF red blood cell (RBC) count may be the only indication that a patient has had an SAH. CT scans are only 90% sensitive for SAH in the acute setting, but a CT scan coupled with an LP increases sensitivity to approximately 98%. It is important in this setting to send both the first and fourth tubes of fluid sample for cell count, because a high RBC count in the first tube that clears by the fourth tube suggests a traumatic LP rather than an SAH. The CSF should also be assessed for xanthochromia (straw color), which indicates a prior SAH (minimum 2–3 days previously) with subsequent RBC lysis, a high protein content, or both.
Many very useful additional specialty tests can be run on CSF. Because they are expensive and often somewhat esoteric, they should only be ordered when clinical circumstances and differential diagnosis evaluation specifically dictate. Among others, these tests include CSF cytology tests for malignant cells; polymerase chain reaction (PCR) tests for tuberculosis (TB) and certain CNS viruses; India ink staining for cryptococcus; antigen titers for viruses and cryptococcus; special TB, fungal, and viral cultures; Venereal Disease Research Laboratories (VDRL) tests for syphilis; immunoglobulin and protein electrophoresis for detecting immune complexes and pathologic proteins; and myelin basic protein and multiple sclerosis testing battery to assess for multiple sclerosis and other demyelinating syndromes.
The LP can be used to introduce dye into the CSF to augment diagnostic studies such as a contrast CT cisternogram for the head or a myelogram or myelogram CT scan for the spine. Myelography was invented by neurosurgery and initially performed predominantly by neurosurgeons. It can also be used therapeutically to relieve ICP by withdrawing CSF on a one-time basis or as a first step in inserting a lumbar drain for chronic spinal CSF withdrawal over days. Care must be taken when contemplating an LP to ensure that the act of LP does not lead to a compartment pressure gradient differential that could lead to herniation and clinical deterioration from a herniation syndrome. The most common scenario is an LP performed in a patient with high ICP due to an intracranial mass lesion leading to either transtentorial or central downward foramen magnum herniation. In general, all patients should be assessed for papilledema with an ophthalmoscope before LP, if possible, and, if doubt persists, a CT or magnetic resonance imaging (MRI) scan should be performed. Rarely, an LP performed below a partial or complete spinal canal CSF block from mass lesion can lead to neurologic deterioration. To rule out spinal canal lesions in patients with a known diagnosis of systemic cancer, an MRI scan of the spine generally should precede diagnostic CSF cytologies performed by LP
Neuroimaging
Before 1976, the only modalities other than the neurologic examination and blood and CSF tests available to the neurosurgeon to diagnose CNS pathology were the cerebral angiogram (dye injected within the cerebral arteries to visualize them on x-ray films), the pneumoencephalogram (air injected into the ventricular system to visualize it on x-ray films), and radionuclide brain scans (injected radioactive tracers sometimes taken up by brain tumors). Both the cerebral angiogram and pneumoencephalogram were invented and were initially performed by neurosurgeons, while the brain scan came from radiology. In 1976, CT revolutionized the ability to see directly brain lesions and brain and skull anatomy. MRI followed in the late 1980s, offering even better resolution of CNS tissue. Few medical disciplines are as critically dependent on imaging for best clinical practice benchmarks as neurosurgery. Neurosurgeons are experienced at interpreting CT and MRI neuroimages and usually work closely with their affiliated neuroradiologist(s).
Currently, nuclear brain scans are rarely performed. The exceptions are to distinguish recurrent primary malignant brain tumor from radiation necrosis after a course of radiation therapy and to trace the flow of CSF after injection of a tracer by LP or CSF device injection. Complete diagnostic pneumoencephalography is no longer performed. However, limited air ventriculography still has a role in acute trauma settings, when the patient is too unstable to go to the CT scanner or has to be taken directly on arrival to surgery for life-saving abdominal or thoracic surgery. In this setting a ventricular catheter can be placed through a burr hole in the skull to serve as an ICP monitor. The catheter can also serve a diagnostic function by using it to withdraw 1 to 2 mL of CSF and then replace it with 1 to 2 mL of air. A subsequent anterior-posterior (A-P) portable skull film will often (but not always) indicate whether midline is shifted to either side and suggest the appropriate side for emergent empiric cranial exploration if clinically indicated.
Cerebral Angiography, Embolization, Thrombolysis, and Embolectomy
Although MR angiography and CT angiography are less invasive and can usually be obtained more quickly, nothing compares with cerebral angiography for detailed resolution of CNS vascular anatomy and definition of CNS vascular lesions. Such lesions include cerebral aneurysm, arteriovenous malformation (AVM), arteriovenous fistula, and arterial embolus. Originally performed by direct needle stick of the cervical CCA and VA, it is now routinely performed by catheters introduced into the femoral artery in the groin and navigated cephalad. Diagnostic cerebral angiography is a very powerful tool; however, it is an invasive test and carries an approximately 1% risk of stroke for anterior circulation assessment, increasing to a 1% to 3% stroke risk for posterior circulation assessment. Diagnostic cerebral angiography can be used to visualize CNS lesions and to assess collateral circulation by assessing the status of the circle of Willis. It can also be used to perform a temporary balloon test occlusion of an artery while the patient is assessed clinically, electrophysiologically, or with CBF imaging.
Cerebral angiography can even be used therapeutically. Endovascular embolization of CNS vascular lesions (e.g., aneurysms and AVMs) was developed by neurosurgeons and is currently performed by both endovascular neurosurgeons as well as interventional radiologists. With endovascular access, selected cases of aneurysms can now be partially or completely occluded by either coil embolization or glue casting. Some AVMs and vascular tumors can now be temporarily (in preparation for microsurgical excision) or permanently embolized with resorbable particles, permanent coils, or casting glue. Endovascular applications of treatment for cerebrovascular disease have now expanded to include angioplasty and stenting for cervical and intracranial carotid, VA disease, and arterial dissections. Intraluminal chemical and mechanical cerebral angioplasty for post-SAH vasospasm, catheter-directed chemical thrombolysis, and endovascular embolus retrieval for stroke are also frequently performed.
CT Scanning
CT scans are the study of choice for neurosurgical emergencies, because they are readily available at most hospitals (even after hours), can be obtained very quickly, and provide a wealth of important information for emergent decision making. They can show bone more clearly than MRI and therefore are excellent when used in evaluating cases for skull fracture. They are very sensitive for detecting acute blood (which appears white), and are therefore useful in assessing patients for SAH, intraparenchymal hemorrhage, hemorrhagic stroke, spontaneous or posttraumatic extra-axial blood clot (epidural or subdural hematoma), or traumatic contusion. They show the ventricular system very well (hypodense) and thus aid in detecting communicating or obstructive hydrocephalus. They can show differentiation between ventricular system displacement and a space-occupying mass lesion. They are useful in detecting abnormal calcium deposits in a blood vessel or brain mass. They are moderately sensitive for detecting swelling or vasogenic edema surrounding a pathologic lesion. CT scan disadvantages include poorer resolution of parenchymal brain structures than MR scanning, less sensitivity for identifying brain tumors (even with intravenous contrast agents), and an inability to show an acute stroke (hypodensity can take 12–36 hours to develop on CT scans). Administration of iodine-based intravenous contrast dye improves the sensitivity of the CT scan for detecting brain tumors and areas of focal infection. Administration of dye into the CSF (CT cisternography) improves its ability to show lesions in the CSF compartments and detect CSF leaks. The development of CT angiography has been a major step forward in assessing suspected SAH for the presence of an aneurysm without the time delay and risks of more invasive cerebral angiography. CT angiography at many centers has now become so good that in some cases neuro-surgeons are able to take patients directly to the operating room to clip an aneurysm microsurgically without ever performing a preoperative cerebral angiogram.
Magnetic Resonanc Imaging
MRI scans do not show bone well, and thus are inferior to CT scanning for assessing patients for fractures or osseus involvement of tumors or infection. Although MRI is very sensitive for detecting subacute or chronic bleeding, it is relatively insensitive compared with CT scanning for detecting acute bleeding and is therefore not the best study for ruling out acute hemorrhage or assessing acute head trauma.
MR scanning provides the best resolution of intracranial soft tissue. Gray matter, white matter distinctions, deep gray matter structures, major cerebral vessels, the meninges, the ventricular lining, and even cranial nerves are very well shown. Flow attenuated inversion-recover (FLAIR) sequences and long time-to-response (TR), or “T2-weighted,” sequences provide excellent sensitivity to even small amounts of vasogenic cerebral edema or demyelination. Gradient response sequences with long TRs provide very good sensitivity to even small amounts of deposited extracellular blood products. Administration of contrast leads to significant improvements in sensitivity over contrast CT scanning for detecting meningeal, ventricular, or cranial nerve pathology, as well as brain tumors and areas of cerebritis and abscess. MRI diffusion studies can almost immediately detect the early cytotoxic edema associated with a stroke, well before the area becomes hypodense on CT scan. As a result, MRI scanning has become the diagnostic procedure of choice for both neurooncology and stroke neurology and for assessing patients for inflammatory lesions and multiple sclerosis. MR angiography also serves as an excellent noninvasive screening tool without iodine dye load for assessing patients for cerebral aneurysm, AVM, atherosclerotic cerebrovascular disease (both cervical and intracranial), and vascular dissection.
Further advances in MRI are rapidly progressing and have exciting potential for clinical neuroscience. High-field 3–tesla magnets have increased soft tissue resolution still further while allowing the high signal-to-noise ratios necessary to perform metabolic tissue sampling through single- and multivoxel MR spectroscopy (MRS). MRS is an excellent technique for distinguishing a mass lesion from tumor, radiation necrosis, infarction, or infection. It can also assess the likelihood of tumor invasion beyond imaging apparent tumor borders into surrounding normally appearing brain. Functional MR can localize sensory and motor speech areas as well as primary motor strip and determine their relationship and degree of direct involvement versus displacement in regard to adjacent mass lesions. Diffusion tensor imaging is a way of mapping the major CNS white matter association tracts (corpus callosum, side-to-side, superior and inferior longitudinal fasciculus, anterior-to-posterior, and the primary motor and sensory long tracts, rostral-to-caudal). It does this by detecting the integrity of selective movement of water within the parallel-oriented cleavage planes along these white matter tract connections. It has the potential for determining whether a mass lesion has invaded and disrupted these cleavage planes within the relevant tracts or merely compressed or displaced them (and if so, demonstrate where they are located relative to the lesion in question). All of this information is very important for clinical decision making and preoperative surgical planning.
Head Injury
Head injury is very common. In the United States, approximately 2 million people each year suffer a head injury and approximately 150,000 people per year become comatose from head injury. Annually, head injury accounts for approximately 1% to 2% of all deaths in the United States, 25% of all trauma-related deaths, and up to 60% of all motor vehicle-related deaths. Head injuries can be acute, subacute, or chronic. Acute head injuries include scalp lacerations, contusions, and abrasions, skull fractures (basal and cranial vault), CSF leakage, extraparenchymal hematomas (subdural and epidural hematomas), brain contusions and intraparenchymal hematomas, traumatic arterial dissection leading to SAH or stroke, and axonal connection shear injuries. Subacute and chronic problems include postconcussive syndrome, chronic subdural hematomas, persistent CSF leak, growing skull fracture, arteriovenous fistulae, infection from retained foreign body, hydrocephalus, posttraumatic epilepsy, and permanent cognitive and neurologic deficits. The initial postresuscitation GCS score is highly predictive of head injury. Patients with GCS scores of 3 to 4 on admission have a 50% to 100% mortality rate. Those with scores of 5 to 6 have a 25% to 65% mortality rate. Patients with scores of 7 to 8 have a 10% to 25% mortality rate. Older patients do worse than younger patients with the same GCS score. Patients who have acute subdural hematomas do worse than other patients with similar GCS scores without subdural hematomas.
Evaluation of Acute Injuries
Evaluation of an acutely head injured patient begins with a primary survey that focuses on the ABCs (airway, breathing, and circulation). Neurologic examination is not reliable or predictive until the patient has been stabilized from the standpoint of ventilation, hypoxemia, and circulatory shock. Careful note should be taken of the need to administer pharmacologic paralytic agents or sedatives during initial resuscitation, because these will significantly affect neurologic assessment. The neurologic assessment begins with a rapid initial survey that determines the patient’s GCS score (Table 8-2), pupillary response, and the symmetry of motor and papillary findings on either side. The degree of impairment and the presence or absence of pending herniation syndrome determines the need to proceed immediately to CT scan for head examination versus the ability to proceed with a secondary neurologic survey before the CT scan. Often, portions outlined in this paragraph are assessed simultaneously or in parallel to save time and expedite the logistics of transport and imaging acquisition. If the patient has a GCS score of 12 or less, the operating room and anesthesiologist should probably be notified to be standing by for possible emergent craniotomy or ICP monitoring procedure.
During the secondary neurologic survey, a careful history from the emergency medical technicians is desirable. The mechanism of injury provides valuable information regarding associated injuries and severity of force transmission to the patient. Whether the patient had a safety belt on, whether the airbag deployed, the structural state of the car, the location the patient was found in the car, and whether the patient was ejected from the car (if the incident was a motor vehicle accident) is very useful information. Knowing whether the patient had a predisposing medical event (e.g., myocardial infarction, drug ingestion) and whether there was a period of hypotension, hypoxemia, or even cardiac arrest is very important. The timing of intubation, if required (in the field versus on arrival), may give valuable clues as to potential secondary hypoxemic injury. During this portion of the evaluation a more detailed systematic neurologic examination is obtained if the patient is conscious and cooperative. If the patient is unconscious or sedated, cranial nerve reflexes (pupils, corneas, gag, and cough) are assessed. The scalp is assessed for signs of bleeding form laceration or abrasion, subgaleal hematoma, projectile wound, or palpable fractures. If an open wound is located, it is noted but is not probed or explored for fear of causing further bleeding and increasing the risk of infection. The patient’s mastoid and periorbital areas are assessed for ecchymosis indicating basilar skull fracture (mastoid—Battle’s sign; periorbital— raccoon eyes). The nose is assessed for signs of CSF rhinorrhea, and, if noted, the trauma surgeon or emergency room physician is instructed (a) to be careful with any subsequent bag-mask pressure ventilation for fear of introducing intracranial air and raising ICP due to ball-valve cranial air entry, and (b) not to insert a nasogastric tube if stomach decompression is required, but to decompress the stomach using an orogastric tube (danger of intracranial penetration by way of an anterior basal skull fracture). The ear canals are examined bilaterally for otorrhea, and the tympanic membranes are assessed for hemotympanum. If the patient is unconscious, has no signs of otorrhea, otorrhagia, or hemotympanum, and if sufficient time is available, caloric testing as an additional brain stem reflex test can be done. Given the risk of cervical spine injury in a patient with an acute head injury, oculocephalic reflex testing is usually deferred until ervical spine injury is excluded.
An unconscious patient should be assessed for history of loss of consciousness, as well as anterograde or retrograde amnesia, which correlate with degree and risk of concussion. Any patient with a history of head injury and a GCS score less than 13 should probably be assessed by CT scan if it is readily available. Patients with GCS score of 13 to 15 can be assessed with CT scan or observed for at least 23 hours (the time a patient can be held in the emergency department before that patient must be admitted to the hospital). Observation consists of repeated neurologic examinations every 2 hours, with a CT scan obtained rapidly if the patient worsens clinically. A patient with a history of a head injury, a GCS score of 15, and a normal neurologic examination has a normal CT scan of the head can probably be discharged home so long as the patient is returning to a stable social situation with an identified responsible adult who has been instructed in how to monitor and assess the patient for the next 23 hours. The patient should return to the hospital if there is hemiparesis, pupillary inequality, increase in headache, sleepiness, decrease in consciousness, or persistent vomiting.
Stabilization
If the patient is exhibiting clinical signs and symptoms of high ICP or herniation syndrome, acute temporizing medical measures to control ICP and maintain CPP should be instituted immediately. Acute or persistent elevations of ICP are treated as described in Table 8-3. Blood pressure and CPP need to be maintained with volume and systemic pressures. In severely injured patients, pharmacologic paralysis with muscle relaxants combined with controlled respiration is necessary to prevent additional increases in ICP because of coughing and straining. Mannitol and furosemide given intravenously are effective in transiently lowering ICP and can be given if the patient’s systemic blood pressure will allow. Hyperventilation does lower ICP, but at the expense of potential ischemia to already injured brain. It is therefore used only on a temporary basis in an acute herniation situation until the cause of the herniation can be identified and rectified or until the ICP can be lowered by pharmacologic or CSF drainage means. Even then, only temporary periods of moderate hyperventilation to CO2 levels of 28 to 30 are recommended. Surgically correctable mass lesions identified on trauma CT scans require urgent neurosurgical intervention if the patient is neurologically compromised and the lesion is sufficiently large. Patients without major surgically reversible mass lesions on CT scan and who have GCS scores of 8 or less are candidates for invasive ICP monitoring as they continue to be treated with neurocritical care measures and assessed for changes in neurologic status by regular examination, ICP and CPP monitoring, and interval neuroimaging reassessment. Careful attention is paid to evaluating the patient’s electrolyte status, serum osmolality, and coagulation status. Intravenous fluids are switched to normal saline from lactated Ringer’s solution as soon as resuscitation of systemic shock is completed.
Once a patient is stabilized but does not improve over time despite normal ICP and CPP, consideration should be given to the possibility of concomitant global ischemic brain injury due to delay in transportation from the scene of injury, severe hypoxemia or hypotension before resuscitation initiation, or delay in some aspect of resuscitation (usually intubation). Another possibility is diffuse axonal shear injury with axonal connection breakage due to lateral shearing forces caused by rapid acceleration–deceleration of the head and intracranial contents. These shear injuries can sometimes be seen on cerebral MRI scans taken several days after the injury. On MRI, they appear to be multiple focal areas of high FLAIR or T2-weighted signal or even multiple areas of petechial hemorrhage on T2-weighted gradient response sequences. They tend to localize to areas of maximal torquing forces (the upper brain stem and diencephalon) and to the point of maximal differential tissue shearing movement (the cortical gray matter–white matter interface). Both conditions leave the patient with a poor prognosis for functional recovery and eventual independence. Any recovery that does occur tends to take place over a long period of time (generally 6–18 months).
Intracranial Pressure Monitoring and Control
In general, monitoring of ICP is indicated for patients with a GCS score of 8 or less. ICP monitors usually take the form of either a ventriculostomy or an intraparenchymal fiberoptic pressure probe. A ventriculostomy consists of a percutaneous Silastic tube inserted through a burr hole in the skull that courses through the brain tissue to the lateral ventricle. The tube provides a continuous column of fluid from the ventricular CSF to an external drainage system and can be transduced like an arterial line. A ventriculostomy is the preferred means of ICP monitoring because it is therapeutic at the same time that it provides the necessary ICP data. Given the conditions of the Monroe-Kellie hypothesis, outlined previously, nothing reduces ICP faster or more effectively than actual CSF drainage. Unfortunately, some ventricular systems are too small to safely intubate, and ventricular chambers can collapse to the point where the ventriculostomy no longer effectively transduces pressure. There are also borderline situations in which the need for ICP monitoring is equivocal or uncertain. In these situations, an intraparenchymal fiberoptic ICP monitor is another option. It provides accurate readings of ICP. However, it cannot drain CSF and becomes increasingly less accurate over time due to zero baseline “drift,” which can gradually lead to inaccuracies of approximately 5 mm Hg over the course of 5 to 10 days. With the ICP known and tracked, the actual CPP for the patient can be determined at any given time (CPP = MAP – ICP), and adjustments made to maintain it on a running basis.
Acute or persistent elevations of ICP are treated as described in Table 8-3. Once CT scanning has ruled out the presence of an acutely reversible surgical mass lesion, and other measures have had a chance to take effect, hyperventilation to even moderate degrees is avoided whenever possible, to prevent secondary ischemic CNS injury. The mainstay of ICP management in the acute head injury situation is CSF drainage through a ventriculostomy. In the presence of reduced brain compliance, even intermittent removal of volumes as small as 1 to 2 mL can have a dramatic and sustained ICP reduction effect. Mannitol is given in intermittent intravenous boluses of 0.125 to 0.25 g/kg (usually 12.5–25 g at a time) every 4 to 6 hours as needed, taking care to follow serum osmolality closely (not allowing it to rise above 315 mOsm/L). The ICP-reducing effects of mannitol can be accentuated by premedication with small intravenous doses of furosemide (10–20 mg/bolus) given 5 to 10 minutes before the mannitol dose. Intravenous infusion sedation with an agent that is short-acting and that can be quickly stopped to allow for neurologic examination (e.g., fentanyl or propofol) will reduce the incidence of struggling against the ventilator, endotracheal tube, or other Valsalva maneuvers, and thus help keep ICP down. Both intravenous sedation and pharmacologic paralysis will reduce muscular tone, thereby increasing venous return from the head and reducing CO2 production from muscle contractions, all of which will help with ICP control. Physiologic levels (up to 5 mm Hg) of positive end expiratory pressure (PEEP) are not likely to have major negative effects on cerebral venous return and ICP, but higher levels of PEEP should be avoided when possible. Serum sodium needs to be carefully monitored because SIADH and resulting hyponatremia can accentuate cerebral edema and ICP problems. Maintenance fluids should consist of normal saline solution with consideration given to judicious use of intravenous 3% NaCl solution if SIADH is confirmed by urine sodium measurement in the presence of low serum sodium (<135 mEq/ L) and elevated ICP. When all else fails, induction of barbiturate coma by bolus injection, followed by continuous infusion, is sometimes necessary. When utilized, the minimum infusion necessary to maintain electrical activity burst-suppression should be confirmed by electroencephalogram (EEG). Induction of barbiturate coma must be considered carefully because it tends to lead to hypothermia, systemic hypotension due to cardiac suppression (which fights against CPP maintenance efforts), and impaired pulmonary ciliary function, which leaves patients susceptible to pneumonia. It also can take some time to wear off.
Cerebral Perfusion Pressure, Tissue Metabolism, Glucose, and Oxygenation
In general, under normal autoregulatory conditions, CBF is not impaired with normal ICP down to MAP as low as 40 mm Hg (CPP ~ 30–32 mm Hg). Even within the globally high ICP situation of pseudotumor cerebri (in which ICP is routinely 27–34 mm Hg), the brain functions normally with a CPP of approximately 36 to 43 mm Hg. However, in head injury, cerebral autoregulation has been shown to be dysfunctional and impaired both globally and in the microenvironment at the site of injury so that CBF can become directly dependent on CPP. Although normal CPP is 50 to 60 mm Hg, in the setting of head injury a desirable target is a minimum CPP of 60 to 70 mm Hg. CPP is maintained by ensuring adequate cardiac preload (CVP 8–10 mm Hg, or pulmonary artery wedge [PAW] pressure 12–15 mm Hg) without volume overload (which could accentuate cerebral edema). Early use of systemic vasopressors is implemented once preload is optimized if a CPP of 60 to 70 mm Hg is not spontaneously achievable. Dopamine has the added advantage of dilating cerebral surface collateral vessels; however, epinephrine or norepinephrine can also be used and may need to be used if dopamine is insufficient.
Another important consideration for maintaining tissue function in the presence of marginal CBF nutrient supply is neuronal metabolic demand. Theoretically, a high metabolic demand situation should lead to greater supply– demand mismatch and potential neuronal injury in the setting of marginal nutrient supply. Given this theoretical observation, efforts are made to control and treat fevers, because elevated temperature increases metabolic demand. Phenytoin is given to prevent seizures, which can markedly increase neuronal metabolic demand and increase ICP. Attempts to purposefully reduce metabolic demand through therapeutic moderate hypothermia have been tried, but have yet to demonstrate improved survival or outcome for major head injury. In addition to reducing ICP, pharmacologically induced barbiturate coma has the added benefit of potentially reducing neuronal metabolic demand and may provide some biochemical free-radical scavenging role at potential sites of continued injury.
In experimental models of CNS ischemia, elevated serum glucose levels before ischemic injury are positively correlated with larger subsequent strokes and worse neurologic outcome. Given the potential role of subsequent ischemia as a major mechanism for secondary CNS injury, glucose levels are carefully controlled and monitored in the patient with head injury. For the first 24 to 48 hours, normal saline solution maintenance fluids do not contain dextrose and the serum glucose level is tightly controlled, even if an insulin infusion is required. This level of tight glucose control continues even after nutrition has been started for the patient and 5% dextrose returned to the intravenous solutions.
Early data suggest that fiberoptic tissue oxygenation probes may prove useful in the management of patients with severe head injury. These fiberoptic probes continuously monitor the extracellular tissue oxygen tension and can be inserted through the same burr hole as a fiberoptic ICP monitor. Responding to low oxygen tension readings with increasing FiO2 has been shown to improve cerebral tissue oxygen tension. Furthermore, it is possible that luxurious cerebral tissue oxygen tension measurements may help identify cases in which moderate hyperventilation (CO2 28–32) may potentially be safely performed, if necessary, to reduce ICP without risking secondary ischemic injury. It should be remembered, however, that these O2 tissue tension monitors only measure local microenvironment oxygenation, which may not reflect oxygenation status at the site of primary and potential secondary injury. Their use has yet to be correlated with improved survival or neurologic outcome from head injury. Other tissue parenchymal monitors such as microdialysis monitors that can measure and follow tissue lactate and amino acid levels are currently being utilized as part of clinical experimental protocols in studying head injury, but have yet to achieve a defined clinical role.
Closed Head Injury
Closed head injury (CHI) describes conditions in which the intradural contents of the cranium are not exposed to the outside environment and are not herniating out through a traumatic cranial defect. CSF leak from a basilar skull fracture is one exception that is still considered a CHI. In the civilian sector, and certainly in times of peace, CHI is the dominant form of head injury seen in the U.S. health care system.
Concussion
Concussion is a clinical syndrome of temporary global brain dysfunction that can be inferred from a history of brief loss of consciousness or a period of anterograde or retrograde amnesia surrounding a CHI. A patient with a history suggestive of a concussion and a history of head injury with a GCS score of 13 to 15 is classified as having a minor closed head injury. Very few of these patients, if imaged, will be found to have a pathologic lesion on CT scan, and the vast majority of these will be nonsurgical in consequence. A concussion does, however, imply significant force transmission to, and absorption by, the brain. Given the delicacy of the CNS, it is not surprising that many of these patients will continue to have annoying symptoms for weeks to months (occasionally for up to 6–12 months after injury) until they finally resolve. The condition is known as postconcussive syndrome. To prevent unwarranted anxiety in patients and assist them in coping with it, counseling should be offered regarding the likelihood of syndrome symptoms. These symptoms include persistent recurring headaches, difficulty concentrating, reduced attention span, short-term memory and learning dysfunction, disturbance in normal wake–sleep rhythms, social disinhibition, emotional lability, depression, and social withdrawal. These symptoms tend to be self-limited and usually resolve given sufficient time.
Closed Skull Fractures
Skull fractures can occur in the skull base or the cranial vault. Skull base fractures involving the anterior cranial fossa may manifest with unilateral or bilateral periorbital swelling and ecchymosis (raccoon eyes). If they involve the orbit roof, floor, and walls, vision acuity and extraocular movements should be carefully checked to rule out extraocular muscle entrapment by the bone fragments. Involvement of the posterior wall of the frontal sinus, cribriform plate, or planum sphenoidale, could indicate risk of early or delayed anterior CSF leak. Anterior cranial fossa skull-base fracture is a relative contraindication for positive pressure bag-mask ventilation and for nasogastric tube placement. The potential risks are intracranial air introduction, elevation in ICP if introduced air cannot subsequently escape (ball-valve mechanism), and intracranial passage of the gastric tube. Basal skull fractures involving the middle cranial fossa are at risk for damaging the ICA in its parasellar/cavernous sinus location. Fractures that extend through the petrous carotid canal exit into the cavernous sinus, or lateral sphenoid sinus wall fractures with sphenoid sinus air-fluid levels may warrant further investigation with CT angiography, MR angiography, or conventional cerebral angiogram. Fractures of the temporal bone can lead to CSF leak through the tympanic membrane (otorrhea) or down the back of the throat via the middle ear and eustachian tube. Visualization of fluid within the mastoid air cells on CT scan should lead to careful search for a temporal bone fracture. Horizontal temporal bone fractures run parallel to the course of the internal auditory canal. Although they can cause CSF leak, they rarely cause cranial nerve dysfunction. Transverse temporal bone fractures run perpendicular to the course of the internal auditory canal. They imply a much larger transmission of force to the skull base and are much more likely than horizontal fractures to be associated with facial, vestibular, and cochlear nerve dysfunction. They are also occasionally associated with mechanical disruption of the middle ear ossicles. Every patient with a temporal bone fracture should be carefully evaluated for facial nerve function, nystagmus, and hearing loss, as well as CSF leak. Fractures that extend through the petrous carotid canal of the temporal bone may warrant further investigation to rule out ICA injury with CT angiography, MR angiography, or conventional cerebral angiogram (see Chapter 5).
Cranial vault fractures can be linear or comminuted (several fragments). They can be diastatic (gap between the fracture edges) or even depressed. Closed cranial vault fractures require surgical exploration only if they are depressed and the depression is greater than the cross-sectional width of the skull (unlikely to heal cosmetically through remodeling alone), if they occur in a cosmetically obvious area (e.g., forehead), if they are associated with an underlying mass lesion that needs to be excised, or are associated with an area of intracranial air suggesting dural penetration. Diastatic fractures in very young children can lead to a special situation called a growing skull fracture. In this situation, dura gets trapped between the edges of the skull fracture and begins to act as a new skull growth plate, preventing proper healing and promoting further diastasis. Growing skull fractures are detected with a 4- to 6-week follow-up skull x-ray and require surgical exploration.
Subdural Hematomas
Subdural hematomas can be acute, subacute, or chronic. Acute subdural hematomas can be spontaneous or traumatic. Subdural hematomas occur between the arachnoid of the brain and the dura of the meninges (Fig. 8-6). They are hyperdense on CT scans (white) due to the presence of fresh blood. On CT scan, they appear to follow the curve of the hemispheric surface like the rind of a watermelon. Spontaneous subdurals usually occur because of tearing of a parasagittal bridging vein due to shearing forces associated with even minor CHIs or cerebral acceleration– deceleration. They are more common in elderly patients who have an accentuated distance between their brain and dura due to cerebral atrophy. They are also more common in patients taking anticoagulant medications (e.g., warfarin, aspirin, dipyridamole, clopidogrel bisulfate). Posttraumatic subdural hematomas are usually associated with an underlying cortical brain injury (e.g., cortical laceration or contusion). As a result, they can be either venous, arterial, or mixed in origin. They carry a significantly worse prognosis than their spontaneous counterparts. Not all subdural hematomas require surgical evacuation. If they are small enough (e.g., <30 mL volume) and the patient is doing well clinically, the patient can be treated conservatively with careful follow-up, neurologic examination, and serial CT scanning. The majority of subdural hematomas will naturally resorb.
Figure 8-6 Magnetic resonance imaging scan of a subacute subdural hematoma.
Within 7 to 14 days the RBCs in the clot begin to lyse and the hematoma may appear as a mixed density mass or even isodense compared with brain. At this subacute stage, the hematoma can sometimes be difficult to see on CT scan, but its presence can usually be detected with careful image windowing, inferred from unilateral loss of gyral surface pattern, or inferred by mass effect on the ipsilateral lateral ventricular. If the hematoma has not been absorbed by approximately 4 to 6 weeks after formation, the body will have walled it off by forming an enclosing membrane of fragile neovascularity, which can easily rebleed with minor trauma. The clot itself is fully lysed, is liquid in consistency (either straw-colored fluid or crankcase-oil appearance grossly, depending on age), and can usually be effectively drained through one or two burr holes.
Epidural hematomas are acute in origin. They occur between the skull and the dura (Fig. 8-5). They are usually associated with adjacent skull fractures. On CT scan they tend to appear biconvex (lenticular) in shape because they can only extend to the next point of dura attachment to the cranial vault (usually a suture line, i.e., coronal or squamosal suture). In adults, fractures of the temporal squama are worrisome because they can be associated with epidural hematomas from damage to the MMA. Given the size of the artery involved and the direct lateral vector the associated clot places on the supratentorial temporal lobe, they are at great risk for rapid enlargement (even in a delayed fashion in a normal-appearing patient) and early herniation syndrome. Fractures in other locations and fractures in the vascular calvarium of children more commonly lead to venous epidural hematomas. Not all epidural hematomas require surgical evacuation. If they are small enough (e.g., <30 mL volume), the patient is doing well clinically. If the location and skull fracture do not suggest MMA involvement, they can be followed conservatively with careful neurologic examination and serial CT scanning. The majority will go on to naturally resorb. Given the fact that the dura remains intact as a protective layer and the fact that they are not routinely associated with underlying direct brain, epidural hematomas carry a much better prognosis than subdural hematomas of the same size and location.
Contusions and Intraparenchymal Hematomas
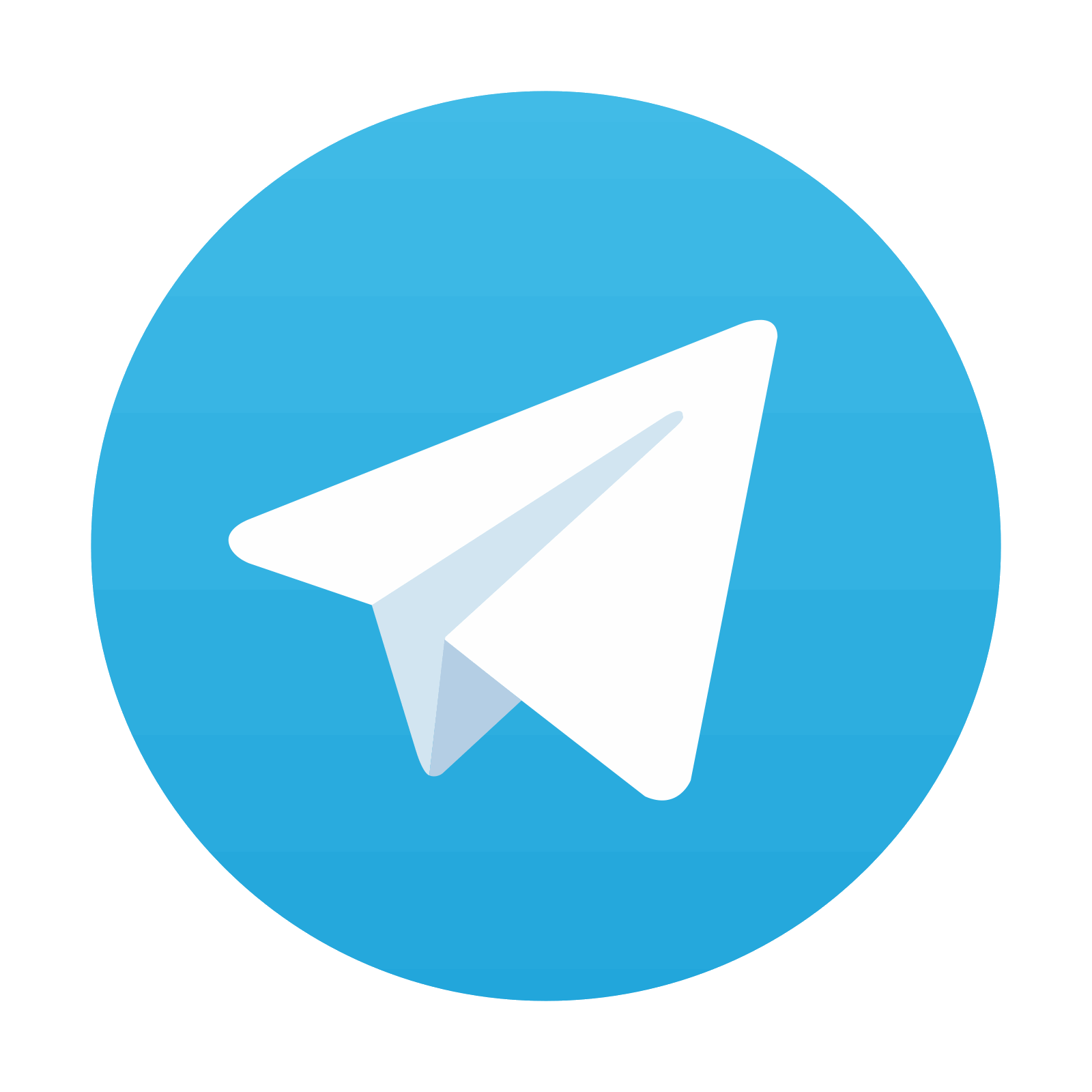
Stay updated, free articles. Join our Telegram channel
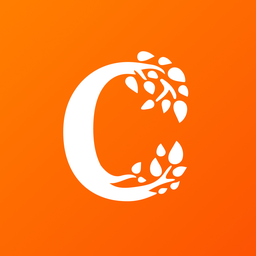
Full access? Get Clinical Tree
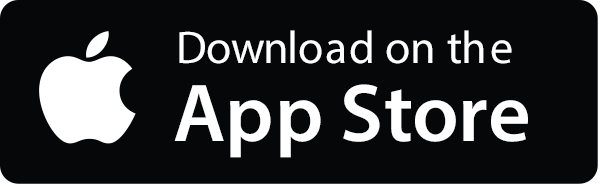
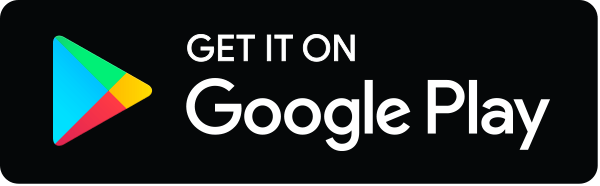