Neurology has long been misperceived as a specialty in which intricate clinical examination and numerous investigations are required to diagnose obscure and untreatable conditions. In fact, it requires careful history-taking with a lesser contribution from targeted examination and considered investigation. The development of specific, effective treatments has made accurate diagnosis essential. Initially, it is important to exclude conditions that constitute neurological emergencies (Box 26.1). If the presentation is not an emergency, more time can be taken to reach a diagnosis. The history should provide a hypothesis for the site and nature of the potential pathology, which a focused examination may refine and inform what further investigation would be useful. A discussion with the patient about possible interventions and rehabilitation may then take place. The nervous system comprises billions of connections between billions of specialised cells, supplied by a complex network of specialised blood vessels. In addition to neurons, there are three types of glial cells. Astrocytes form the structural framework for neurons and control their biochemical environment. Astrocyte foot processes are intimately associated with blood vessels, forming the blood–brain barrier (Fig. 26.1). Oligodendrocytes are responsible for the formation and maintenance of the myelin sheath, which surrounds axons and is essential for the rapid transmission of action potentials by saltatory conduction. Microglial cells derive from monocytes/macrophages and play a role in fighting infection and removing damaged cells. Peripheral neurons have axons invested in myelin made by Schwann cells. Ependymal cells line the cerebral ventricles. The role of the central nervous system (CNS) is to generate outputs in response to external stimuli and changes in internal conditions. Each neuron receives input by synaptic transmission from dendrites (branched projections of other neurons), which may sum to produce output in the form of an action potential. This is conducted down axons, with synaptic transmission to other neurons or, in the motor system, to muscle cells. These processes require the maintenance of an electrochemical gradient across neuron cell membranes by specialised membrane ion channels. Synaptic transmission involves the release of neurotransmitters that modulate the function of the target cell by interacting with structures on the cell surface, including ion channels and other cell surface receptors (Fig. 26.2). At least 20 different neurotransmitters are known to act at different sites in the nervous system, and all are potentially amenable to pharmacological manipulation. Major components of the nervous system and their interrelationships are depicted in Figure 26.3. The cerebral hemispheres coordinate the highest level of nervous function, the anterior half dealing with executive (‘doing’) functions and the posterior half constructing a perception of the environment. Each cerebral hemisphere has four functionally specialised lobes (Fig. 26.4 and Box 26.2), but some functions are lateralised, and this depends on cerebral dominance (i.e. the hemisphere in which language is represented). Cerebral dominance aligns limb dominance with language function: in right-handed individuals the left hemisphere is almost always dominant, while around half of left-handers have a dominant right hemisphere. The parietal lobes integrate sensory perception. The primary sensory cortex lies in the post-central gyrus of the parietal lobe. Much of the remainder is devoted to ‘association’ cortex, which processes and interprets input from the various sensory modalities. The supramarginal and angular gyri of the dominant parietal lobe form part of the language area (p. 1169). Close to these are regions dealing with numerical function. The non-dominant parietal lobe is concerned with spatial awareness and orientation. CSF is formed in the lateral ventricles and protects and nourishes the CNS. The CSF flows from third to fourth ventricles and through foramina in the brainstem to dissipate over the surface of the CNS, eventually being reabsorbed into the cerebral venous system (see Fig. 26.41, p. 1217). In addition to containing all the sensory and motor pathways entering and leaving the hemispheres, the brainstem houses the nuclei and projections of the cranial nerves, as well as other important collections of neurons in the reticular formation (Fig. 26.5). Cranial nerve nuclei provide motor control to muscles of the head (including the face and eyes) and coordinate sensory input from the special sense organs and the face, nose, mouth, larynx and pharynx. They also relay autonomic messages, including pupillary, salivary and lacrimal functions. The reticular formation is predominantly involved in the control of conjugate eye movements, the maintenance of balance, cardiorespiratory control and the maintenance of arousal. A programme of movement formulated by the pre-motor cortex is converted into a series of signals in the motor cortex that are transmitted to the spinal cord in the pyramidal tract (Fig. 26.6). This passes through the internal capsule and the ventral brainstem before decussating in the medulla to enter the lateral columns of the spinal cord. The pyramidal tract ‘upper motor neurons’ synapse with the anterior horn cells of the spinal cord grey matter, which form the lower motor neurons. Lower motor neurons in the anterior horn of the spinal cord innervate a group of muscle fibres termed a ‘motor unit’. Loss of lower motor neurons causes loss of contraction within this unit, resulting in weakness and reduced muscle tone. Subsequently, denervated muscle fibres atrophy, causing muscle wasting, and depolarise spontaneously, causing ‘fibrillations’. Except in the tongue, these are usually only perceptible on electromyelography (EMG; p. 1152). With the passage of time, neighbouring intact neurons sprout to provide re-innervation, but the neuromuscular junctions of the enlarged motor units are unstable and depolarise spontaneously, causing fasciculations (which are large enough to be visible to the naked eye). Fasciculations therefore imply chronic partial denervation with re-innervation. Circuits between the basal ganglia and the motor cortex constitute the extrapyramidal system, which controls muscle tone, body posture and the initiation of movement (see Fig. 26.6). Lesions of the extrapyramidal system produce an increase in tone that, unlike spasticity, is continuous throughout the range of movement at any speed of stretch (‘lead pipe’ rigidity). Involuntary movements are also a feature of extrapyramidal lesions (p. 1165), and tremor in combination with rigidity produces typical ‘cogwheel’ rigidity. Extrapyramidal lesions also cause slowed and clumsy movements (bradykinesia), which characteristically reduce in size with repetition, as well as postural instability, which can precipitate falls. The neurological organisation of visual pathways is shown in Figure 26.7. Fibres from ganglion cells in the retina pass to the optic disc and then backwards through the lamina cribrosa to the optic nerve. Nasal optic nerve fibres (subserving the temporal visual field) cross at the chiasm, but temporal fibres do not. Hence, fibres in each optic tract and further posteriorly carry representation of contralateral visual space. From the lateral geniculate nucleus, lower fibres pass through the temporal lobes on their way to the primary visual area in the occipital cortex, while the upper fibres pass through the parietal lobe. Normally, the eyes move conjugately (in unison), though horizontal convergence allows visual fusion of objects at different distances. The control of eye movements begins in the cerebral hemispheres, particularly within the frontal eye fields, and the pathway then descends to the brainstem with input from the visual cortex, superior colliculus and cerebellum. Horizontal and vertical gaze centres in the pons and mid-brain, respectively, coordinate output to the ocular motor nerve nuclei (3, 4 and 6), which are connected to each other by the medial longitudinal fasciculus (MLF) (Fig. 26.8). The MLF is particularly important in coordinating horizontal movements of the eyes. The extraocular muscles are then supplied by the oculomotor (3rd), trochlear (4th) and abducens (6th) cranial nerves. Much of the cerebral cortex is involved in the process of forming and interpreting communicating sounds, especially in the dominant hemisphere (see Box 26.2, p. 1142). Decoding of speech sounds (phonemes) is carried out in the upper part of the posterior temporal lobe. The attribution of meaning, as well as the formulation of the language required for the expression of ideas and concepts, occurs predominantly in the lower parts of the anterior parietal lobe (the angular and supramarginal gyri). The temporal speech comprehension region is referred to as Wernicke’s area (Fig. 26.9). Other parts of the temporal lobe contribute to verbal memory, where lexicons of meaningful words are ‘stored’. Parts of the non-dominant parietal lobe appear to contribute to non-verbal aspects of language in recognising meaningful intonation patterns (prosody). Sensory information from the limbs ascends the nervous system in two anatomically discrete systems (Fig. 26.10). Fibres from proprioceptive organs and those mediating well-localised touch (including vibration) enter the spinal cord at the posterior horn and pass without synapsing into the ipsilateral posterior columns. Neural fibres conveying pain and temperature sensory information (nociceptive neurons) synapse with second-order neurons that cross the midline in the spinal cord before ascending in the contralateral anterolateral spinothalamic tract to the brainstem. Pain is a complex percept that is only partly related to activity in nociceptor neurons (Fig. 26.11). In the posterior horn of the spinal cord, the second-order neuron of the spinothalamic tract is affected by a number of influences in addition to its synapse with the fibres from nociceptors. Branches from the larger mechanoceptor fibres destined for the posterior column also synapse with the second-order spinothalamic neurons and with interneurons of the grey matter of the posterior horn. The nociceptor neurons release neurotransmitters (such as substance P), in addition to excitatory transmitters, which influence the excitability of the spinothalamic neurons. Activity in the posterior horn neurons is modulated by fibres descending from the peri-aqueductal grey matter of the mid-brain and raphe nuclei of the medulla. Neurons of this ‘descending analgesia system’ are activated by endogenous opiate (endorphin) peptides. The spinal cord’s posterior horn is therefore much more than a relay station in pain transmission; its complexity allows it to ‘gate’ and modulate painful sensation before it ascends in the spinothalamic tract. In the diencephalon, the perception of pain is further influenced by the rich interconnections of the thalamus with the limbic system. The physiology and pathology of mood disorders are discussed elsewhere (Ch. 10) but it is important to remember that any process affecting brain function will have some effect on mood and affect. Conversely, mood disorder will have a significant effect on perception and function. It can be difficult to disentangle whether psychological and psychiatric changes are the cause or the effect of any neurological symptoms. After taking a history and examining the patient, the clinician should have an idea of the nature and site of any pathology. Given the density of tracts and nuclei in the brainstem (see Fig. 26.5), detailed localisation may be possible on the basis of history and examination alone, to be confirmed or refuted by investigation. For example, in a patient presenting with sudden onset of upper motor neuron features affecting the right face, arm and leg in association with a left 3rd nerve palsy, the lesion will be in the left cerebral peduncle in the brainstem and the pathology is likely to have been a small stroke, as the onset was sudden. This combination of signs is known as Weber’s syndrome, and is one of several well-described brainstem syndromes, which are listed in Box 26.3. The effects of individual cranial nerve deficits are discussed in the sections on eye movements (p. 1169) and on facial weakness, sensory loss in brainstem lesions, dysphonia and dysarthria, and bulbar symptoms (pp. 1163, 1165, 1168 and 1173). Neurological imaging has traditionally allowed assessment of structure only. Various techniques are available, including X-rays (plain X-rays, computed tomography (CT), CT angiography, myelography and angiography), magnetic resonance (MR imaging (MRI), MR angiography (MRA)) and ultrasound (Doppler imaging of blood vessels). The uses and limitations of each of these are shown in Box 26.4. Examples of brain imaged by the various techniques are shown in Figure 26.12. Plain X-rays are useful in the investigation of trauma to vertebrae, but their value in providing information about non-bony tissues is limited, which makes them far less helpful in the assessment of inflammatory and degenerative conditions of the spine. MRI has transformed the investigation of these areas, since it can give information not only about the vertebrae and intervertebral discs, but also about their effects on the spinal cord and nerve roots. Myelography is an invasive technique involving injection of contrast into the lumbar theca. While the outline of the nerve roots and spinal cord provides information about abnormal structure, the accuracy and wide availability of MRI have reduced the need for this. Myelography may still be used for technical reasons or where MRI is unavailable, contraindicated, or precluded by the patient’s claustrophobia. Examples of the cervical spine imaged by plain X-rays, myelography and MRI are shown in Figure 26.13. Abnormalities in the EEG result from a number of conditions. Examples include an increase in fast frequencies (beta) seen with sedating drugs such as benzodiazepines, or marked focal slowing noted over a structural lesion such as a tumour or an infarct. Improved quality and accessibility of imaging have made EEG redundant in lesion localisation, except in the specialist investigation of epilepsy (p. 1182). EEG remains useful in progressive and continuous disorders such as reduced consciousness (p. 1159), in encephalitis (p. 1205), and in certain dementias such as Creutzfeldt–Jakob disease (p. 1211). The EEG in epilepsy is predominantly used in its classification and prognosis, and in some patients to localise the seat of epileptiform discharges when surgery is being considered. During an epileptic seizure, high-voltage disturbances of background activity (‘discharges’) will often be noted. These may be generalised, as in the 3-Hz ‘spike and wave’ of childhood absence epilepsy, or more focal, as in localisation-related epilepsies (Fig. 26.14). Techniques such as hyperventilation or photic stimulation can be used to increase the yield of epileptiform changes, particularly in the generalised epilepsy syndromes. While some argue that it is possible to detect ‘spikes’ and ‘sharp waves’ to lend support to a clinical diagnosis, these are non-specific and therefore not diagnostic. Electrical stimulation of a nerve causes an impulse to travel both efferently and afferently along the underlying axons. Nerve conduction studies (NCS) make use of this, recording action potentials as they pass along peripheral nerves and (with motor nerves) as they pass into the muscle belly. Digital recording has enhanced sensitivity and reproducibility of these tiny potentials. By measuring the time taken to traverse a known distance, it is possible to calculate nerve conduction velocities (NCVs). Healthy nerves at room temperature will conduct at a speed of 40–50 m/s. If the recorded potential is smaller than expected, this provides evidence of a reduction in the overall number of functioning axons. Significant slowing of conduction velocity, in contrast, suggests impaired saltatory conduction due to peripheral nerve demyelination. Such changes in NCS may be diffuse (as in a hereditary demyelinating peripheral neuropathy, p. 1223), focal (as in pressure palsies, p. 1224) or multifocal (e.g. Guillain–Barré syndrome, p. 1224; mononeuritis multiplex, p. 1224). The information gained can allow the disease responsible for peripheral nerve dysfunction to be better deduced (see Box 26.99, p. 1223). Stimulation of motor nerves allows for the recording of compound muscle action potentials (CMAPs) over muscles (Fig. 26.15). These are around 500 times larger than sensory nerve potentials, typically around 1–20 millivolts. Since a proportion of stimulated impulses in motor nerves will ‘reflect’ back to the anterior horn cell body (forming the ‘F’ wave), it is also possible to obtain some information about the condition of nerve roots. Repetitive nerve stimulation (RNS) at 3–15/s provides consistent CMAPs in healthy muscle. In myasthenia gravis (p. 1226), where there is partial blockage of acetylcholine receptors, however, there is a diagnostic fall (decrement) in CMAP amplitude. In contrast, an increasing CMAP with high-frequency RNS is seen in Lambert–Eaton myasthenic syndrome (p. 1227). The cortical response to visual, auditory or electrical stimulation can be measured on an EEG as an evoked potential (EP). If a stimulus is provided – for example, to the eye – the tiny EEG response can be discerned when averaging 100–1000 repeated stimuli. Assessing the latency (the time delay) and amplitude can give information about the integrity of the relevant pathway. MRI now provides more information about CNS pathways, thus reducing reliance on EPs. In practice, visual evoked potentials (VEPs) are most commonly used to help differentiate CNS demyelination from small-vessel white-matter changes (Fig. 26.16).
Neurological disease
Clinical examination of the nervous system
Functional anatomy and physiology
Cells of the nervous system
Generation and transmission of the nervous impulse
(1) An action potential arriving at the nerve terminal depolarises the membrane and this opens voltage-gated calcium channels. (2) Entry of calcium causes the fusion of synaptic vesicles containing neurotransmitters with the pre-synaptic membrane and release of the neurotransmitter across the synaptic cleft. (3) The neurotransmitter binds to receptors on the post-synaptic membrane either (A) to open ligand-gated ion channels which, by allowing ion entry, depolarise the membrane and initiate an action potential (4), or (B) to bind to metabotrophic receptors that activate an effector enzyme (e.g. adenylyl cyclase) and thus modulate gene transcription via the intracellular second messenger system, leading to changes in synthesis of ion channels or modulating enzymes. (5) Neurotransmitters are taken up at the pre-synaptic membrane and/or metabolised.
(cAMP = cyclic adenosine monophosphate; DNA = deoxyribonucleic acid; mRNA = messenger ribonucleic acid)
Functional anatomy of the nervous system
Cerebral hemispheres
(1) CSF is synthesised in the choroid plexus of the ventricles, and flows from the lateral and third ventricles through the aqueduct to the fourth ventricle. (2) At the foramina of Luschka and Magendie it exits the brain, flowing over the hemispheres (3) and down around the spinal cord and roots in the subarachnoid space. (4) It is then absorbed into the dural venous sinuses via the arachnoid villi.
The brainstem
The motor system
Neurons from the motor cortex descend as the pyramidal tract in the internal capsule and cerebral peduncle to the ventral brainstem, where most cross low in the medulla (A). In the spinal cord the upper motor neurons form the corticospinal tract in the lateral column before synapsing with the lower motor neurons in the anterior horns. The activity in the motor cortex is modulated by influences from the basal ganglia and cerebellum. Pathways descending from these structures control posture and balance (B).
Lower motor neurons
The extrapyramidal system
Vision
Schematic representation of eyes and brain in transverse section.
Downward projections pass from the cortex to the pontine lateral gaze centre (A). The pontine gaze centre projects to the 6th cranial nerve nucleus (B), which innervates the ipsilateral lateral rectus and projects to the contralateral 3rd nerve nucleus (and hence medial rectus) via the medial longitudinal fasciculus (MLF). Tonic inputs from the vestibular apparatus (C) project to the contralateral 6th nerve nucleus via the vestibular nuclei.
Speech
The somatosensory system
Pain
Personality and mood
Localising lesions in the brainstem
Investigation of neurological disease
Neuroimaging
Head and orbit
A Skull X-ray showing lytic skull lesion (eosinophilic granuloma – arrow). B CT showing complete middle cerebral artery infarct (arrows). C MRI showing widespread areas of high signal in multiple sclerosis (arrows). D SPECT after caudate infarct showing relative hypoperfusion of overlying right cerebral cortex (arrows).
Cervical, thoracic and lumbar spine
A Lateral X-ray showing bilateral C6/7 facet dislocation. B Myelogram showing widening of cervical cord due to astrocytoma (arrows). C MRI showing posterior epidural compression from adenocarcinomatous metastasis to the posterior arch of T1 (arrows).
Neurophysiological testing
Electroencephalography
A Generalised epileptic discharge, as seen in epilepsy syndromes such as childhood absence or juvenile myoclonic epilepsy. B Focal sharp waves over the right parietal region (circled), with spread of discharge to cause a generalised tonic–clonic seizure.
Nerve conduction studies
Electrodes (R) on the muscle (abductor pollicis here) record the compound muscle action potential (CMAP) after stimulation at the median nerve at the wrist (S1) and from the elbow (S2). The velocity from elbow to wrist can be determined if the distance between the two stimulating electrodes (d) is known. A prolonged L1 (L = latency) would be caused by dysfunction distally in the median nerve (e.g. in carpal tunnel syndrome). A prolonged L2 is caused by slow nerve conduction (as in demyelinating neuropathy). The F wave is a small delayed response that appears when the electrical signal travels backwards to the anterior horn cell, sparking a second action potential in a minority of fibres (see text).
(NCV = nerve conduction velocity)
Evoked potentials
Stay updated, free articles. Join our Telegram channel
Full access? Get Clinical Tree
Neurological disease
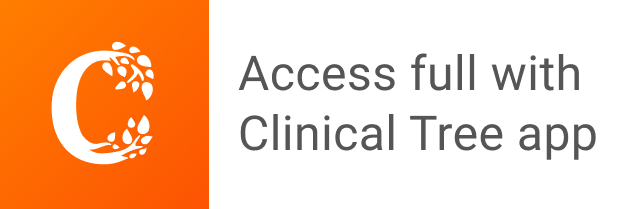