Nervous System Cells
ORGANIZATION OF THE NERVOUS SYSTEM
The nervous system is organized to detect changes (stimuli) in the internal and external environment, evaluate that information, and possibly respond by initiating changes in muscles or glands. To make this complex network of information lines and processing circuits easier to understand, biologists have subdivided the nervous system into the smaller “systems” and “divisions” described in the following paragraphs and illustrated in Figure 13-2. Note as you read through the next several sections that the nervous system can be divided in various ways: according to structure, direction of information flow, or control of effectors.
Central and Peripheral Nervous Systems
The classical manner of subdividing the nervous system is based on the gross dissections of early anatomists. It simply categorizes all nervous system tissues according to their relative positions in the body: central or peripheral.
The central nervous system (CNS) is, as its name implies, the structural and functional center of the entire nervous system. Consisting of the brain and spinal cord, the CNS integrates incoming pieces of sensory information, evaluates the information, and initiates an outgoing response. Today, neurobiologists include only those cells that begin and end within the anatomical boundaries of the brain and spinal cord as part of the CNS. Cells that begin in the brain or cord but extend out through a nerve are thus not included in the central nervous system.
The peripheral nervous system (PNS) consists of the nerve tissues that lie in the periphery, or “outer regions,” of the nervous system. Nerves that originate from the brain or exit through the skull are called cranial nerves, and nerves that originate from the spinal cord and do not exit the skull are called spinal nerves.
The terms central and peripheral are often used as directional terms in the nervous system. For example, nerve cell extensions called nerve fibers may be called central fibers if they extend from the cell body toward the CNS. Likewise, they may be called peripheral fibers if they extend from the cell body away from the CNS.
Figure 13-1 represents the anatomical components of the CNS and PNS and their relative positions in the body. Figure 13-2 represents the relationship of the CNS and PNS in diagram form.
Afferent and Efferent Divisions
The tissues of both the central and the peripheral nervous systems include nerve cells that form incoming information pathways and outgoing pathways. For this reason, it is often convenient to categorize the nervous pathways into divisions according to the direction in which they carry information. The afferent division of the nervous system consists of all of the incoming sensory or afferent pathways. The efferent division of the nervous system consists of all the outgoing motor or efferent pathways. The literal meanings of the terms afferent (carry toward) and efferent (carry away) may help you distinguish between these two divisions of the nervous system more easily.
Look at Figure 13-2 and try to distinguish the afferent and efferent pathways represented there. Here, and throughout the rest of this book, afferent pathways are typically represented in blue and efferent pathways in red.
Somatic and Autonomic Nervous Systems
Yet another way to organize the components of the nervous system for ease of study is to categorize them according to the type of effectors they regulate. Some pathways of the somatic nervous system (SNS) carry information to the somatic effectors, which are the skeletal muscles. These motor pathways make up the somatic motor division. As Figure 13-2 shows, the somatic nervous system also includes the afferent pathways, making up the somatic sensory division, that provide feedback from the somatic effectors. The SNS also includes the integrating centers that receive the sensory information and generate the efferent response signal.
Efferent pathways of the autonomic nervous system (ANS) carry information to the autonomic, or visceral, effectors, which are mainly the smooth muscles, cardiac muscle, glands, adipose tissue, and other “involuntary” tissue. As its name implies, the autonomic nervous system seems autonomous of voluntary control—it usually appears to govern itself without our conscious knowledge. We now know that the autonomic nervous system is influenced by the conscious mind, but the historical name for this part of the nervous system has remained.
The efferent pathways of the ANS can be divided into the sympathetic division and the parasympathetic division. The sympathetic division, made up of pathways that exit the middle portions of the spinal cord, is involved in preparing the body to deal with immediate threats to the internal environment. It produces the “fight-or-flight” response. The parasympathetic pathways exit at the brain or lower portions of the spinal cord and coordinate the body’s normal resting activities. The parasympathetic division is thus sometimes called the “rest-and-repair” division.
The afferent pathways of the ANS belong to the visceral sensory division, which carries feedback information to the autonomic integrating centers in the central nervous system.
Figure 13-2 summarizes the various ways in which the nervous system is subdivided and combines these approaches into a single “big picture.”
CELLS OF THE NERVOUS SYSTEM
Two main types of cells compose the nervous system, namely, neurons and glia. Neurons are excitable cells that conduct the impulses that make possible all nervous system functions. In other words, they form the “wiring” of the nervous system’s information circuits. Glia, or glial cells, on the other hand, do not usually conduct information themselves but support the function of neurons in various ways. Some of the major types of glia and neurons are described in the following sections.
Glia
Our understanding of glia, or neuroglia as they are sometimes called, has been slow in coming. In the late nineteenth century the Italian cell biologist Camillo Golgi (after whom the Golgi apparatus is named) accidentally dropped a piece of brain tissue in a bath of silver nitrate. When he finally found it, Golgi could see a vast network of various kinds of darkly stained cells surrounding the neurons—proof that glia existed. However, they were almost immediately set aside as mere packing material. In fact, glia literally means “glue.” For more than a century almost all research efforts focused on neurons. Fortunately, the tide has turned, and studies of glia and their functions are one of the hottest areas in neurobiology, the study of the nervous system. We are now finding that they have a major role in how the nervous system works.
The number of glia in the human nervous system is beyond imagination. One estimate places the figure at a staggering 900 billion, or nine times the estimated number of stars in our galaxy! Unlike some neurons, glial cells retain their capacity for cell division throughout adulthood. Although this characteristic gives them the ability to replace themselves, it also makes them susceptible to abnormalities of cell division—such as cancer. Most benign and malignant tumors found in the nervous system originate in glial cells.
As stated earlier, glia serve various roles in supporting the function of neurons. To get a sense of this variety of functions, we shall briefly examine five major types of glia (Figure 13-3):
The first four glial types in this list are located in the CNS. Only the Schwann cells are located in the PNS.
The star-shaped glia, astrocytes (Figure 13-3, A), derive their name from the Greek astron, “star.” Found only in the central nervous system, they are the largest and most numerous type of glia. Their long, delicate “points” extend through brain tissue, attaching to both neurons and the tiny blood capillaries of the brain. Astrocytes have recently been called “stars of the nervous system” because of the many important functions they perform. Astrocytes actually “feed” the neurons by picking up glucose from the blood, converting it to lactic acid, and passing it along to the neurons to which they are connected. (See Chapter 30 for a fuller discussion of the role of lactic acid in cellular respiration.)
Because webs of astrocytes form tight sheaths around the brain’s blood capillaries, they help form the blood-brain barrier (BBB). The BBB is a double barrier made up of astrocyte “feet” and the endothelial cells that make up the walls of the capillaries. Small molecules (e.g., oxygen, carbon dioxide, water, alcohol) diffuse rapidly through the barrier to reach brain neurons and other glia. Larger molecules penetrate it slowly or not at all (Box 13-1). More recent findings suggest that astrocytes may not only influence the growth of neurons and how the neurons connect to form circuits but also transmit information along “astrocyte pathways” themselves.
Microglia (Figure 13-3, B) are small, usually stationary cells found in the central nervous system. In inflamed or degenerating brain tissue, however, microglia enlarge, move about, and carry on phagocytosis. In other words, they engulf and destroy microorganisms and cellular debris. Although classified as glia, microglia are functionally and developmentally unrelated to other nervous system cells.
Ependymal cells (Figure 13-3, C) are glia that resemble epithelial cells, forming thin sheets that line fluid-filled cavities in the brain and spinal cord. Some ependymal cells take part in producing the fluid that fills these spaces. Other ependymal cells have cilia that help keep the fluid circulating within the cavities.
Oligodendrocytes (Figure 13-3, D) are smaller than astrocytes and have fewer processes. The name oligodendrocytes literally means “cell with few branches” (oligo- few, -dendro- branch, -cyte cell). Some oligodendrocytes lie clustered around nerve cell bodies; and some are arranged in rows between nerve fibers in the brain and cord. They help hold nerve fibers together and also serve another and probably more important function—they produce the fatty myelin sheath around nerve fibers in the central nervous system (Box 13-2).
Notice in Figure 13-3, D, how their processes wrap around surrounding nerve fibers to form this sheath.
Schwann cells (Figure 13-3, E to G) are found only in the peripheral nervous system. Here they serve as the functional equivalent of the oligodendrocytes, supporting nerve fibers and sometimes forming a myelin sheath around them. As Figure 13-3, F, shows, many Schwann cells can wrap themselves around a single nerve fiber. The myelin sheath is formed by layers of Schwann cell membrane containing the white, fatty substance myelin. Microscopic gaps in the sheath, between adjacent Schwann cells, are called nodes of Ranvier or simply myelin sheath gaps. The myelin sheath and its tiny gaps are important in the proper conduction of impulses along nerve fibers in the peripheral nervous system. As you can see in Figure 13-4, the developing Schwann cell wraps around the nerve fiber in a way that forms an inner core of many layers of plasma membrane, made up mostly of the myelin (a type of phospholipid). Notice that the Schwann cell’s nucleus and cytoplasm are squeezed to the perimeter to form the neurilemma. The neurilemma is essential to normal nerve growth and the regeneration of injured nerve fibers. Schwann cells are also called neurolemmocytes. The myelin sheath along with the neurilemma is sometimes called the neuronal sheath.
Figure 13-3, E, shows that some Schwann cells do not wrap around nerve fibers to form a thick myelin sheath but simply hold fibers together in a bundle. Nerve fibers with many Schwann cells forming a thick myelin sheath are called myelinated fibers, or white fibers. When several nerve fibers are held by a single Schwann cell that does not wrap around them to form a thick myelin sheath, the fibers are called unmyelinated fibers, or gray fibers.
Figure 13-3, G, shows a type of Schwann cell often called a satellite cell. Like satellites positioned around a planet, these special Schwann cells surround the cell body of a neuron. Satellite cells support neuronal cell bodies in regions called ganglia in the peripheral nervous system.
Neurons
The human brain is estimated to contain almost 100 billion neurons, or about half of the total number of nervous system cells in the brain. All neurons consist of a cell body (also called the perikaryon, or soma) and at least two processes: one axon and one or more dendrites (Figure 13-5). Because dendrites and axons are threadlike extensions from a neuron’s cell body, they are often called nerve fibers.
In many respects the cell body, the largest part of a nerve cell, resembles other cells. It contains a nucleus, cytoplasm, and various organelles found in other cells, for example, mitochondria and a Golgi apparatus. The location of the nucleus in the cell body not only makes it easy to find in a microscopic specimen but also provides the name perikaryon (literally, “surrounding the nucleus”). A neuron’s cytoplasm extends through its cell body and its processes. A plasma membrane encloses the entire neuron.
In the cell body, rough endoplasmic reticulum (ER) and its attached ribosomes provide protein molecules for the neuron. Some of these proteins are then processed and packaged into vesicles by the Golgi apparatus. Some protein molecules in these vesicles are needed for the transmission of nerve signals from one neuron to another. Such proteins are called neurotransmitters. Other proteins are used in the maintenance and repair of the neuron.
The cell body also contains many mitochondria, which replicate themselves in the cell body. Some of the resulting mitochondria are transported to the end of the axon to provide energy (adenosine triphosphate [ATP]) for nerve signaling there.
Dendrites usually branch extensively from the cell body—like tiny trees. In fact, their name derives from the Greek word for tree. The distal ends of dendrites of sensory neurons may be called receptors because they receive the stimuli that initiate nerve signals. Some dendrites in the brain have small knoblike dendritic spines, which serve as connection points for other neurons. Dendrites receive stimuli and conduct electrical signals toward the cell body and/or axon of the neuron.
The axon of a neuron is a single process that usually extends from a tapered portion of the cell body called the axon hillock. Axons conduct impulses away from the cell body. Although a neuron has only one axon, that axon often has one or more side branches, called axon collaterals. Moreover, the distal tips of axons form branches called telodendria that each terminate in a synaptic knob (see Figure 13-5). Each synaptic knob contains mitochondria and numerous vesicles.
Some axons have varicosities, or swellings, which act as points of contact with other cells such as smooth muscle fibers (see Figure 12-31 on p. 371). Axons vary in both length and diameter. Some are a meter long. Some, however, measure only a few millimeters. Axon diameters also vary considerably, from about 20 μm down to about 1 μm—a point of interest because axon diameter relates to velocity of impulse conduction. In general, the larger the diameter, the more rapid the conduction.
Whether an axon is myelinated or not also affects the speed of impulse conduction. Figure 13-6 shows a cross section of a typical myelinated axon. Notice in the figure how a series of Schwann cells have grown over the axon in a spiral fashion to form the myelin sheath and neurilemma (see also Figure 13-4). Only axons may have a myelin sheath—dendrites do not. The role of the myelin sheath and nodes of Ranvier in impulse conduction is discussed later.
Extending through the cytoplasm of each neuron are fine strands sometimes called neurofibrils (see Figure 13-6). Neurofibrils are bundles of intermediate filaments called neurofilaments. Microtubules and microfilaments are additional components of the neuron’s cytoskeleton. Along with providing structural support, a neuron’s cytoskeleton forms a sort of “railway” for the rapid transport of small organelles to and from the far ends of a neuron. Figure 13-7 shows how small “motor molecules” attach to mitochondria and vesicles containing neurotransmitters and carry them to the end of the axon. The “used” vesicles and transmitters are then returned to the cell body by the same process, but in reverse, for recycling. This type of movement is called axonal transport.
Figure 13-8 summarizes the different functional regions of the neurons, based on their role in receiving and conducting nerve signals. The dendrites and cell body act primarily as an input zone, receiving nerve stimulation and initiating nerve impulses in response. The axon hillock acts as a summation zone by adding together all the nerve impulses arriving from the cell body and dendrites—and deciding whether to send the impulse any farther along the neuron. The axon is the conduction zone because its primary job is to conduct the nerve impulse from the axon hillock all the way to the end of the neuron. The telodendria of the axon, along with their synaptic knobs, together act as an output zone where vesicles of neurotransmitter are released for possible reception by a nearby neuron or effector cell (muscle or gland cell).
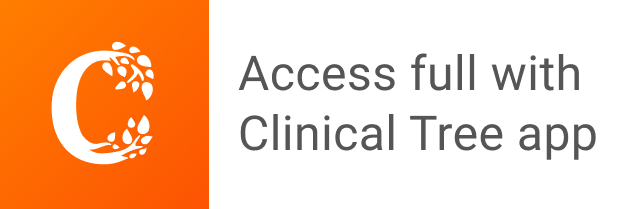