49
Muscle & the Cytoskeleton
OBJECTIVES
After studying this chapter, you should be able to:
Understand the general biochemical features of skeletal, cardiac, and smooth muscle contraction.
Know the biologic effects of nitric oxide (NO).
Indicate the different metabolic fuels required for a sprint and for the marathon.
Know the general structures and functions of the major components of the cytoskeleton, namely microfilaments, microtubules, and intermediate filaments.
Understand the bases of malignant hyperthermia Duchenne and Becker muscular dystrophies, inherited cardiomyopathies, the Hutchinson-Gilford syndrome (progeria), and several skin diseases due to abnormal keratins.
BIOMEDICAL IMPORTANCE
Proteins play an important role in movement at both the organ (eg, skeletal muscle, heart, and gut) and cellular levels. In this chapter, the roles of specific proteins and certain other key molecules (eg, Ca2+) in muscular contraction are described. A brief coverage of cytoskeletal proteins is also presented.
Knowledge of the molecular bases of a number of conditions that affect muscle has advanced greatly in recent years. Understanding of the molecular basis of Duchenne-type muscular dystrophy was greatly enhanced when it was found that it was due to mutations in the gene encoding dystrophin (see case history no. 7 in Chapter 57). Significant progress has also been made in understanding the molecular basis of malignant hyperthermia, a serious complication for some patients undergoing certain types of anesthesia. Heart failure is a very common medical condition, with a variety of causes; its rational therapy requires understanding of the biochemistry of heart muscle. One group of conditions that cause heart failure are the cardiomyopathies, some of which are genetically determined. NO has been found to be a major regulator of smooth muscle tone. Many widely used vasodilators— such as nitroglycerin, used in the treatment of angina pectoris—act by increasing the formation of NO. Muscle, partly because of its mass, plays major roles in the overall metabolism of the body.
MUSCLE TRANSDUCES CHEMICAL ENERGY INTO MECHANICAL ENERGY
Muscle is the major biochemical transducer (machine) that converts potential (chemical) energy into kinetic (mechanical) energy. Muscle, the largest single tissue in the human body, makes up somewhat less than 25% of body mass at birth, more than 40% in the young adult, and somewhat less than 30% in the aged adult. We shall discuss aspects of the three types of muscles found in vertebrates: skeletal, cardiac, and smooth. Both skeletal and cardiac muscles appear striated upon microscopic observation; smooth muscle is nonstriated. Although skeletal muscle is under voluntary nervous control, the control of both cardiac and smooth muscle is involuntary.
Sarcoplasm of Muscle Cells Contains ATP, Phosphocreatine, & Glycolytic Enzymes
Striated muscle is composed of multinucleated muscle fiber cells surrounded by an electrically excitable plasma membrane, the sarcolemma. An individual muscle fiber cell, which may extend the entire length of the muscle, contains a bundle of many myofibrils arranged in parallel, embedded in intracellular fluid termed sarcoplasm. Within this fluid is contained glycogen, the high-energy compounds ATP and phosphocreatine, and the enzymes of glycolysis.
Sarcomere Is the Functional Unit of Muscle
An overall view of voluntary muscle at several levels of organization is presented in Figure 49–1.
FIGURE 49–1 The structure of voluntary muscle. The sarcomere is the region between the Z lines. (Drawing by Sylvia Colard Keene. Reproduced, with permission, from Bloom W, Fawcett DW: A Textbook of Histology, 10th ed. Saunders, 1975.)
When the myofibril is examined by electron microscopy, alternating dark and light bands (anisotropic bands, meaning birefringent in polarized light, and isotropic bands, meaning not altered by polarized light) can be observed. These bands are thus referred to as A and I bands, respectively. The central region of the A band (the H band) appears less dense than the rest of the band. The I band is bisected by a very dense and narrow Z line (Figure 49–2).
FIGURE 49–2 Arrangement of filaments in striated muscle. (A) Extended. The positions of the I, A, and H bands in the extended state are shown. The thin filaments partly overlap the ends of the thick filaments, and the thin filaments are shown anchored in the Z lines (often called Z disks). In the lower part of Figure 49–2(A), “arrowheads,” pointing in opposite directions, are shown emanating from the myosin (thick) filaments. Four actin (thin) filaments are shown attached to two Z lines via α-actinin. The central region of the three myosin filaments, free of arrowheads, is called the M band (not labeled). Cross sections through the M bands, through an area where myosin and actin filaments overlap and through an area in which solely actin filaments are present, are shown. (B) Contracted. The actin filaments are seen to have slipped along the sides of the myosin fibers toward each other. The lengths of the thick filaments (indicated by the A bands) and the thin filaments (distance between Z lines and the adjacent edges of the H bands) have not changed. However, the lengths of the sarcomeres have been reduced (from 2300 to 1500 nm), and the lengths of the H and I bands are also reduced because of the overlap between the thick and thin filaments. These morphologic observations provided part of the basis for the sliding filament model of muscle contraction.
The sarcomere is defined as the region between two Z lines (Figures 49-1 and 49-2) and is repeated along the axis of a fibril at distances of 1500-2300 nm depending upon the state of contraction.
The striated appearance of voluntary and cardiac muscle in light microscopic studies results from their high degree of organization, in which most muscle fiber cells are aligned so that their sarcomeres are in parallel register (Figure 49–1).
Thick Filaments Contain Myosin; Thin Filaments Contain Actin, Tropomyosin & Troponin
When myofibrils are examined by electron microscopy, it appears that each one is constructed of two types of longitudinal filaments. One type, the thick filament, confined to the A band, contains chiefly the protein myosin. These filaments are about 16 nm in diameter and arranged in the cross-section as a hexagonal array (Figure 49–2, center; right-hand cross-section).
The thin filament (about 7 nm in diameter) lies in the I band and extends into the A band but not into its H zone (Figure 49–2). Thin filaments contain the proteins actin, tropomyosin, and troponin (Figure 49–3). In the A band, the thin filaments are arranged around the thick (myosin) filament as a secondary hexagonal array. Each thin filament lies symmetrically between three thick filaments (Figure 49–2, center, mid cross section), and each thick filament is surrounded symmetrically by six thin filaments.
FIGURE 49–3 Schematic representation of the thin filament, showing the spatial configuration of its three major protein components: actin, myosin, and tropomyosin. The upper panel shows individual molecules of G-actin. The middle panel shows actin monomers assembled into F-actin. Individual molecules of tropomyosin (two strands wound around one another) and of troponin (made up of its three subunits) are also shown. The lower panel shows the assembled thin filament, consisting of F-actin, tropomyosin, and the three subunits of troponin (TpC, Tpl, and TpT).
The thick and thin filaments interact via cross bridges that emerge at intervals of 14 nm along the thick filaments. As depicted in Figure 49–2, the cross bridges (drawn as arrowheads at each end of the myosin filaments, but not shown extending fully across to the thin filaments) have opposite polarities at the two ends of the thick filaments. The two poles of the thick filaments are separated by a 150-nm segment (the M band, not labeled in the figure) that is free of projections.
The Sliding Filament Cross-Bridge Model Is the Foundation on Which Current Thinking About Muscle Contraction Is Built
This model was proposed independently in the 1950s by Henry Huxley and Andrew Huxley and their colleagues. It was largely based on careful morphologic observations on resting, extended, and contracting muscle. Basically, when muscle contracts, there is no change in the lengths of the thick and thin filaments, but the H zones and the I bands shorten (see legend to Figure 49–2). Thus, the arrays of inter-digitating filaments must slide past one another during contraction. Cross-bridges that link thick and thin filaments at certain stages in the contraction cycle generate and sustain the tension. The tension developed during muscle contraction is proportionate to the filament overlap and to the number of cross bridges. Each cross-bridge head is connected to the thick filament via a flexible fibrous segment that can bend outward from the thick filament. This flexible segment facilitates contact of the head with the thin filament when necessary but is also sufficiently pliant to be accommodated in the interfilament spacing.
ACTIN & MYOSIN ARE THE MAJOR PROTEINS OF MUSCLE
The mass of a muscle is made up of 75% water and more than 20% protein. The two major proteins are actin and myosin.
Monomeric G-actin (43 kDa; G, globular) makes up 25% of muscle protein by weight. At physiologic ionic strength and in the presence of Mg2+, G-actin polymerizes noncovalently to form an insoluble double helical filament called F-actin (Figure 49–3). The F-actin fiber is 6-7 nm thick and has a pitch or repeating structure every 35.5 nm.
Myosins constitute a family of proteins, with at least 12 classes having been identified in the human genome. The myosin discussed in this chapter is myosin-II, and when myosin is referred to in this text, it is this species that is meant unless otherwise indicated. Myosin-I is a monomeric species that binds to cell membranes. It may serve as a linkage between microfilaments and the cell membrane in certain locations.
Myosin contributes 55% of muscle protein by weight and forms the thick filaments. It is an asymmetric hexamer with a molecular mass of approximately 460 kDa. Myosin has a fibrous tail consisting of two intertwined helices. Each helix has a globular head portion attached at one end (Figure 49–4). The hexamer consists of one pair of heavy (H) chains each of approximately 200 kDA molecular mass, and two pairs of light (L) chains each with a molecular mass of approximately 20 kDa. The L chains differ, one being called the essential light chain and the other the regulatory light chain. Skeletal muscle myosin binds actin to form actomyosin (actin-myosin), and its intrinsic ATPase activity is markedly enhanced in this complex. Isoforms of myosin exist whose amounts can vary in different anatomic, physiologic, and pathologic situations.
FIGURE 49–4 Diagram of a myosin molecule showing the two intertwined α-helices (fibrous portion), the globular region or head (G), the light chains (L), and the effects of proteolytic cleavage by trypsin and papain. The globular region (myosin head) contains an actin-binding site and an L chain-binding site and also attaches to the remainder of the myosin molecule.
The structures of actin and of the head of myosin have been determined by X-ray crystallography; these studies have confirmed a number of earlier findings concerning their structures and have also given rise to much new information.
Limited Digestion of Myosin with Proteases Has Helped to Elucidate Its Structure & Function
When myosin is digested with trypsin, two myosin fragments (meromyosins) are generated. Light meromyosin (LMM) consists of aggregated, insoluble α-helical fibers from the tail of myosin (Figure 49–4). LMM exhibits no ATPase activity and does not bind to F-actin.
Heavy meromyosin (HMM; molecular mass about 340 kDa) is a soluble protein that has both a fibrous portion and a globular portion (Figure 49–4). It exhibits ATPase activity and binds to F-actin. Digestion of HMM with papain generates two subfragments, S-1 and S-2. The S-2 fragment is fibrous in character, has no ATPase activity, and does not bind to F-actin.
S-1 (molecular mass approximately 115 kDa) does exhibit ATPase activity, binds L chains, and in the absence of ATP will bind to and decorate actin with “arrowheads” (Figure 49–5). Both S-1 and HMM exhibit ATPase activity, which is accelerated 100- to 200-fold by complexing with F-actin. As discussed below, F-actin greatly enhances the rate at which myosin ATPase releases its products, ADP and Pi. Thus, although F-actin does not affect the hydrolysis step per se, its ability to promote release of the products produced by the ATPase activity greatly accelerates the overall rate of catalysis.
FIGURE 49–5 The decoration of actin filaments with the S-1 fragments of myosin to form “arrowheads.” (Courtesy of JA Spudich.)
CHANGES IN THE CONFORMATION OF THE HEAD OF MYOSIN DRIVE MUSCLE CONTRACTION
How can hydrolysis of ATP produce macroscopic movement? Muscle contraction essentially consists of the cyclic attachment and detachment of the S-1 head of myosin to the F-actin filaments. This process can also be referred to as the making and breaking of cross-bridges. The attachment of actin to myosin is followed by conformational changes that are of particular importance in the S-1 head and are dependent upon which nucleotide is present (ADP or ATP). These changes result in the power stroke, which drives movement of actin filaments past myosin filaments. The energy for the power stroke is ultimately supplied by ATP, which is hydrolyzed to ADP and Pi. However, the power stroke itself occurs as a result of conformational changes in the myosin head when ADP leaves it.
The major biochemical events occurring during one cycle of muscle contraction and relaxation can be represented in the five steps shown in Figure 49–6 as follows:
FIGURE 49–6 The hydrolysis of ATP drives the cyclic association and dissociation of actin and myosin in five reactions described in the text. (Modified, with permission, from Stryer L: Biochemistry, 2nd ed. Freeman, 1981. Copyright © 1981 by W. H. Freeman and Company.)
1. In the relaxation phase of muscle contraction, the S-1 head of myosin hydrolyzes ATP to ADP and Pi, but these products remain bound. The resultant ADP-Pi-myosin complex has been energized and is in a so-called high-energy conformation.
2. When contraction of muscle is stimulated (via events involving Ca2+, troponin, tropomyosin, and actin, which are described below), actin becomes accessible and the S-1 head of myosin finds it, binds it, and forms the actin-myosin-ADP-i complex indicated.
3. Formation of this complex promotes the release of Pi, which initiates the power stroke. This is followed by release of ADP and is accompanied by a large conformational change in the head of myosin in relation to its tail (Figure 49–7), pulling actin about 10 nm toward the center of the sarcomere. This is the power stroke. The myosin is now in a so-called low-energy state, indicated as actinmyosin.
FIGURE 49–7 Representation of the active cross bridges between thick and thin filaments. This diagram was adapted by AF Huxley from HE Huxley: the mechanism of muscular contraction. Science 1969;164:1356. The latter proposed that the force involved in muscular contraction originates in a tendency for the myosin head (S-1) to rotate relative to the thin filament and is transmitted to the thick filament by the S-2 portion of the myosin molecule acting as an inextensible link. Flexible points at each end of S-2 permit S-1 to rotate and allow for variations in the separation between filaments. This figure is based on HE Huxley’s proposal, but also incorporates elastic (the coils in the S-2 portion) and stepwise-shortening elements (depicted here as four sites of interaction between the S-1 portion and the thin filament). (See Huxley AF, Simmons RM: Proposed mechanism of force generation in striated muscle. Nature [Lond] 1971;233:533.) The strengths of binding of the attached sites are higher in position 2 than in position 1 and higher in position 3 than position 2. The myosin head can be detached from position 3 with the utilization of a molecule of ATP; this is the predominant process during shortening. The myosin head is seen to vary in its position from about 90° to about 45°, as indicated in the text. (S-1, myosin head; S-2, portion of the myosin molecule; LMM) (see legend to Figure 49–4). (Reproduced from Huxley AF: Muscular contraction. J Physiol 1974;243:1. By kind permission of the author and the Journal of Physiology.)
4. Another molecule of ATP binds to the S-1 head, forming an actinmyosin-ATP complex.
5. Myosin-ATP has a low affinity for actin, and actin is thus released. This last step is a key component of relaxation and is dependent upon the binding of ATP to the actinmyosin complex.
Another cycle then commences with the hydrolysis of ATP (step 1 of Figure 49–6), re-forming the high-energy conformation.
Thus, hydrolysis of ATP is used to drive the cycle, with the actual power stroke being the conformational change in the S-1 head that occurs upon the release of ADP. The hinge regions of myosin (referred to as flexible points at each end of S-2 in the legend to Figure 49–7) permit the large range of movement of S-1 and also allow S-1 to find actin filaments.
If intracellular levels of ATP drop (eg, after death), ATP is not available to bind the S-1 head (step 4 above), actin does not dissociate, and relaxation (step 5) does not occur. This is the explanation for rigor mortis, the stiffening of the body that occurs after death.
Calculations have indicated that the efficiency of contraction is about 50%; that of the internal combustion engine is less than 20%.
Tropomyosin & the Troponin Complex Present in Thin Filaments Perform Key Functions in Striated Muscle
In striated muscle, there are two other proteins that are minor in terms of their mass but important in terms of their function. Tropomyosin is a fibrous molecule that consists of two chains, alpha and beta, that attach to F-actin in the groove between its filaments (Figure 49–3). Tropomyosin is present in all muscular and muscle-like structures. The troponin complex is unique to striated muscle and consists of three polypeptides. Troponin T (TpT) binds to tropomyosin as well as to the other two troponin components. Troponin I (TpI) inhibits the F-actin-myosin interaction and also binds to the other components of troponin. Troponin C (TpC) is a calcium-binding polypeptide that is structurally and functionally analogous to calmodulin, an important calcium-binding protein widely distributed in nature. Four molecules of calcium ion are bound per molecule of troponin C or calmodulin, and both molecules have a molecular mass of 17 kDa.
Ca2+ Plays a Central Role in Regulation of Muscle Contraction
The contraction of muscles from all sources occurs by the general mechanism described above. Muscles from different organisms and from different cells and tissues within the same organism may have different molecular mechanisms responsible for the regulation of their contraction and relaxation. In all systems, Ca2+ plays a key regulatory role. There are two general mechanisms of regulation of muscle contraction: actin-based and myosin-based. The former operates in skeletal and cardiac muscles, the latter in smooth muscle.
Actin-Based Regulation Occurs in Striated Muscle
Actin-based regulation of muscle occurs in vertebrate skeletal and cardiac muscles, both striated. In the general mechanism described above (Figure 49–6), the only potentially limiting factor in the cycle of muscle contraction might be ATP. The skeletal muscle system is inhibited at rest; this inhibition is relieved to activate contraction. The inhibitor of striated muscle is the troponin system, which is bound to tropomyosin and F-actin in the thin filament (Figure 49–3). In striated muscle, there is no control of contraction unless the tropomyosin-troponin systems are present along with the actin and myosin filaments. As described above, tropomyosin lies along the groove of F-actin, and the three components of troponin— TpT, TpI, and TpC—are bound to the F-actin-tropomyosin complex. TpI prevents binding of the myosin head to its Factin attachment site either by altering the conformation of F-actin via the tropomyosin molecules or by simply rolling tropomyosin into a position that directly blocks the sites on F-actin to which the myosin heads attach. Either way prevents activation of the myosin ATPase that is mediated by binding of the myosin head to F-actin. Hence, the TpI system blocks the contraction cycle at step 2 of Figure 49–6. This accounts for the inhibited state of relaxed striated muscle.
The Sarcoplasmic Reticulum Regulates Intracellular Levels of Ca2+ in Skeletal Muscle
In the sarcoplasm of resting muscle, the concentration of Ca2+ is 10-8 to 10-7 mol/L. The resting state is achieved because Ca2+ is pumped into the sarcoplasmic reticulum (SR) through the action of an active transport system, called the Ca2+ ATPase (Figure 49–8), initiating relaxation. The SR is a network of fine membranous sacs. Inside the SR, Ca2+ is bound to a specific Ca2+-binding protein-designated calsequestrin. The sarcomere is surrounded by an excitable membrane (the T-tubule system) composed of transverse (T) channels closely associated with the SR.
FIGURE 49–8 Diagram of the relationships among the sarcolemma (plasma membrane), a T tubule, and two cisternae of the SR of skeletal muscle (not to scale). The T tubule extends inward from the sarcolemma. A wave of depolarization, initiated by a nerve impulse, is transmitted from the sarcolemma down the T tubule. It is then conveyed to the Ca2+ release channel (RYR), perhaps by interaction between it and the dihydropyridine receptor (slow Ca2+ voltage channel), which are shown in close proximity. Release of Ca2+ from the Ca2+ release channel into the cytosol initiates contraction. Subsequently, Ca2+ is pumped back into the cisternae of the SR by the Ca2+ ATPase (Ca2+ pump) and stored there, in part bound to calsequestrin.
When the sarcolemma is excited by a nerve impulse, the signal is transmitted into the T-tubule system and a Ca2+ release channel in the nearby SR opens, releasing Ca2+ from the SR into the sarcoplasm. The concentration of Ca2+ in the sarcoplasm rises rapidly to 10-5 mol/L. The Ca2+-binding sites on TpC in the thin filament are quickly occupied by Ca2+. The TpC-4Ca2+ interacts with TpI and TpT to alter their interaction with tropomyosin. Accordingly, tropomyosin moves out of the way or alters the conformation of F-actin so that the myosin head-ADP-Pi (Figure 49–6) can interact with F-actin to start the contraction cycle.
The Ca2+ release channel is also known as the ryanodine receptor (RYR). There are two isoforms of this receptor, RYR1 and RYR2, the former being present in skeletal muscle and the latter in heart muscle and brain. Ryanodine is a plant alkaloid that binds to RYR1 and RYR2 specifically and modulates their activities. The Ca2+ release channel is a homotetramer made up of four subunits of kDa 565. It has transmembrane sequences at its carboxyl terminal, and these probably form the Ca2+ channel. The remainder of the protein protrudes into the cytosol, bridging the gap between the SR and the transverse tubular membrane. The channel is ligand-gated, Ca2+ and ATP working synergistically in vitro, although how it operates in vivo is not clear. A possible sequence of events leading to opening of the channel is shown in Figure 49–9. The channel lies very close to the dihydropyridine receptor (DHPR), a voltage-dependent calcium channel of the transverse tubule system (Figure 49–8). Experiments in vitro employing an affinity column chromatography approach have indicated that a 37-amino-acid stretch in RYR1 interacts with one specific loop of DHPR.
FIGURE 49–9 Possible chain of events leading to opening of the Ca2+ release channel. As indicated in the text, the Ca2+ voltage channel and the Ca2+ release channel have been shown to interact with each other in vitro via specific regions in their polypeptide chains. (DHPR, dihydropyridine receptor; RYR1, RYR 1.)
Relaxation occurs when sarcoplasmic Ca2+ falls below 10-7 mol/L owing to its resequestration into the SR by Ca2+ ATPase. TpC-4Ca2+ thus loses its Ca2+. Consequently, roponin, via interaction with tropomyosin, inhibits further myosin head and F-actin interaction, and in the presence of ATP the myosin head detaches from the F-actin.
Thus, Ca2+ controls skeletal muscle contraction and relaxation by an allosteric mechanism mediated by TpC, TpI, TpT, tropomyosin, and F-actin.
A decrease in the concentration of ATP in the sarcoplasm (eg, by excessive usage during the cycle of contraction-relaxation or by diminished formation, such as might occur in ischemia) has two major effects: (1) The Ca2+ ATPase (Ca2+ pump) in the SR ceases to maintain the low concentration of Ca2+
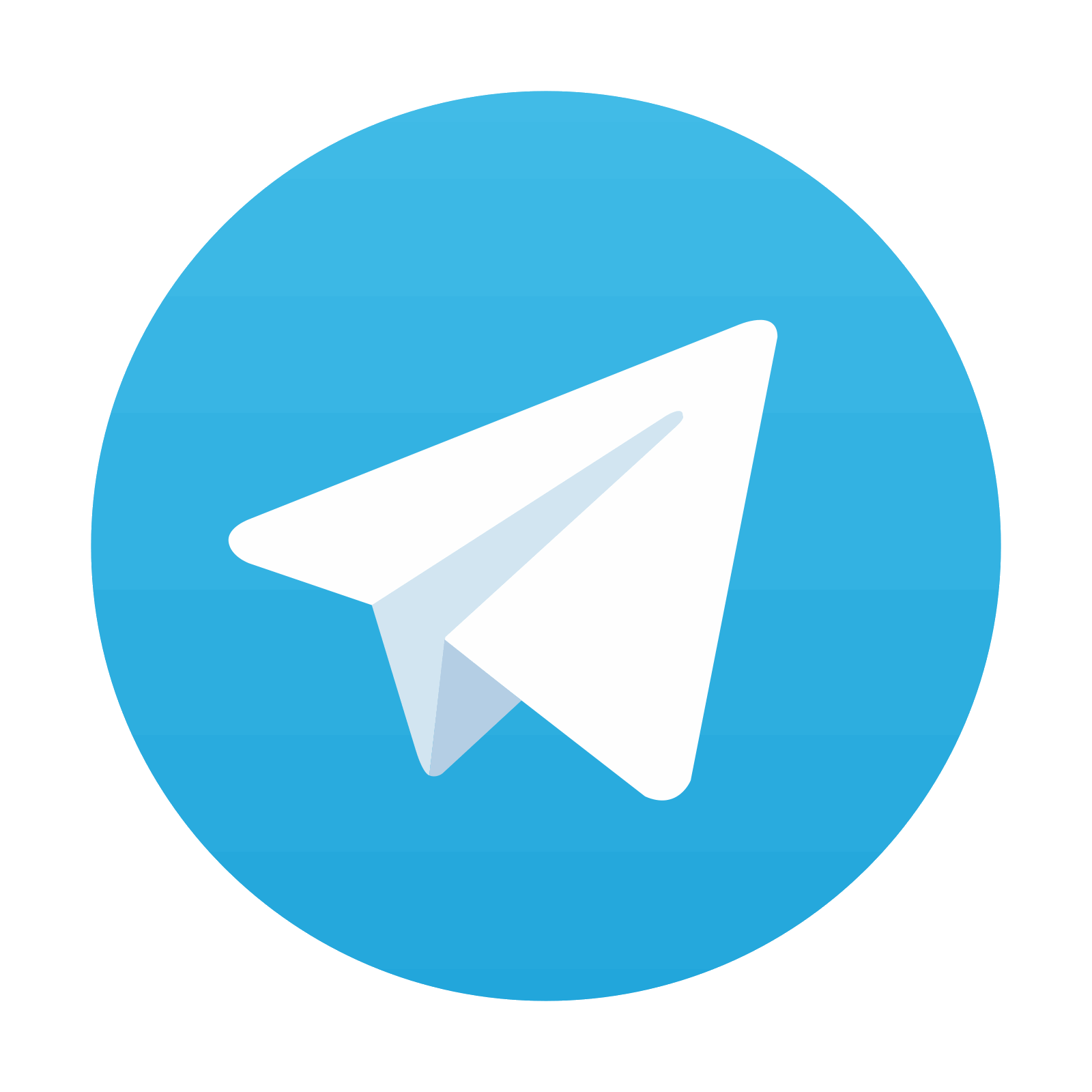
Stay updated, free articles. Join our Telegram channel
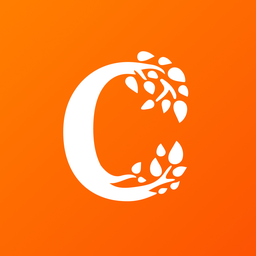
Full access? Get Clinical Tree
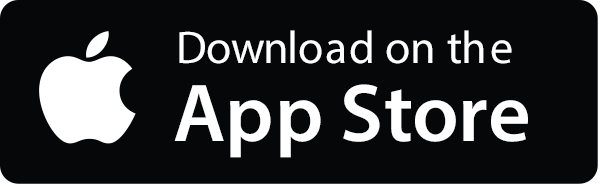
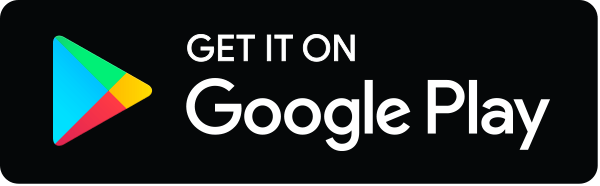