33
Metabolism of Purine & Pyrimidine Nucleotides
OBJECTIVES
After studying this chapter, you should be able to:
Compare and contrast the roles of dietary nucleic acids and of de novo biosynthesis in the production of purines and pyrimidines destined for polynucleotide biosynthesis.
Explain why antifolate drugs and analogs of the amino acid glutamine inhibit purine biosynthesis.
Outline the sequence of reactions that convert IMP, first to AMP and GMP, and subsequently to their corresponding nucleoside triphosphates.
Describe the formation from ribonucleotides of deoxyribonucleotides (dNTPs).
Indicate the regulatory role of PRPP in hepatic purine biosynthesis and the specific reaction of hepatic purine biosynthesis that is feedback inhibited by AMP and by GMP.
State the relevance of coordinated control of purine and pyrimidine nucleotide biosynthesis.
Identify reactions that are inhibited by anticancer drugs.
Write the structure of the end product of purine catabolism. Comment on its solubility and indicate its role in gout, Lesch-Nyhan syndrome, and von Gierke disease.
Identify reactions whose impairment leads to modified pathologic signs and symptoms.
Indicate why there are few clinically significant disorders of pyrimidine catabolism.
BIOMEDICAL IMPORTANCE
Even when humans consume a diet rich in nucleoproteins, dietary purines and pyrimidines are not incorporated directly into tissue nucleic acids. Humans synthesize the nucleic acids, ATP, NAD+, coenzyme A, etc, from amphibolic intermediates. However, injected purine or pyrimidine analogs, including potential anticancer drugs, may be incorporated into DNA. The biosyntheses of purine and pyrimidine ribonucleotide triphosphates (NTPs) and dNTPs are precisely regulated events. Coordinated feedback mechanisms ensure their production in appropriate quantities and at times that match varying physiologic demand (eg, cell division). Human diseases that involve abnormalities in purine metabolism include gout, Lesch-Nyhan syndrome, adenosine deaminase deficiency, and purine nucleoside phosphorylase deficiency. Diseases of pyrimidine biosynthesis are rarer, but include orotic acidurias. Unlike the low solubility of uric acid formed by catabolism of purines, the end-products of pyrimidine catabolism (carbon dioxide, ammonia, β-alanine, and γ-aminoisobutyrate) are highly water soluble. One genetic disorder of pyrimidine catabolism is β-hydroxybutyric aciduria, due to total or partial deficiency of the enzyme dihydropyrimidine dehydrogenase. This disorder of pyrimidine catabolism, also known as combined uraciluria-thyminuria, is also a disorder of β-amino acid biosynthesis, since the formation of β-alanine and of β-aminoisobutyrate is impaired. A nongenetic form can be triggered by administration of 5-fluorouracil to patients with low levels of dihydropyrimidine dehydrogenase.
PURINES & PYRIMIDINES ARE DIETARILY NONESSENTIAL
Normal human tissues can synthesize purines and pyrimidines from amphibolic intermediates in quantities and at times appropriate to meet variable physiologic demand. Ingested nucleic acids and nucleotides therefore are dietarily nones-sential. Following their degradation in the intestinal tract, the resulting mononucleotides may be absorbed or converted to purine and pyrimidine bases. The purine bases are then oxidized to uric acid, which may be absorbed and excreted in the urine. While little or no dietary purine or pyrimidine is incorporated into tissue nucleic acids, injected compounds are incorporated. The incorporation of injected [3H]thymidine into newly synthesized DNA thus can be used to measure the rate of DNA synthesis.
BIOSYNTHESIS OF PURINE NUCLEOTIDES
With the exception of parasitic protozoa, all forms of life synthesize purine and pyrimidine nucleotides. Synthesis from amphibolic intermediates proceeds at controlled rates appropriate for all cellular functions. To achieve homeostasis, intracellular mechanisms sense and regulate the pool sizes of NTPs, which rise during growth or tissue regeneration when cells are rapidly dividing.
Purine and pyrimidine nucleotides are synthesized in vivo at rates consistent with physiologic need. Early investigations of nucleotide biosynthesis first employed birds, and later Escherichia coli. Isotopic precursors of uric acid fed to pigeons established the source of each atom of a purine (Figure 33–1) and initiated study of the intermediates of purine biosynthesis. Avian tissues also served as a source of cloned genes that encode enzymes of purine biosynthesis and the regulatory proteins that control the rate of purine biosynthesis.
FIGURE 33–1 Sources of the nitrogen and carbon atoms of the purine ring. Atoms 4, 5, and 7 (blue highlight) derive from glycine.
The three processes that contribute to purine nucleotide biosynthesis are, in order of decreasing importance.
1. Synthesis from amphibolic intermediates (synthesis de novo).
2. Phosphoribosylation of purines.
3. Phosphorylation of purine nucleosides.
INOSINE MONOPHOSPHATE (IMP) IS SYNTHESIZED FROM AMPHIBOLIC INTERMEDIATES
Figure 33–2 illustrates the intermediates and the 11 enzyme-catalyzed reactions that convert α-D-ribose 5-phosphate to inosine monophosphate (IMP). The first intermediate formed in the de novo pathway for purine biosynthesis is 5-phosphoribosyl 5-pyrophosphate (PRPP; structure II, Figure 33–2). PRPP is also an intermediate in the biosynthesis of pyrimidine nucleotides, NAD+, and NADP+. Separate branches then lead from IMP to AMP and GMP (Figure 33–3). Subsequent phosphoryl transfer from ATP converts AMP and GMP to ADP and GDP. Conversion of GDP to GTP involves a second phosphoryl transfer from ATP, whereas conversion of ADP to ATP is achieved primarily by oxidative phosphorylation (see Chapter 13).
FIGURE 33–2 Purine biosynthesis from ribose 5-phosphate and ATP. See the text for explanations.
FIGURE 33–3 Conversion of IMP to AMP and GMP.
Multifunctional Catalysts Participate in Purine Nucleotide Biosynthesis
In prokaryotes, each reaction of Figure 33–2 is catalyzed by a different polypeptide. By contrast, the enzymes of eukaryotes are polypeptides that possess multiple catalytic activities whose adjacent catalytic sites facilitate channeling of intermediates between sites. Three distinct multifunctional enzymes catalyze reactions ,
, and
; reactions
and
; and reactions
and
of Figure 33–2.
Antifolate Drugs and Glutamine Analogs Block Purine Nucleotide Biosynthesis
The carbons added in reactions and
of Figure 33–2 are contributed by derivatives of tetrahydrofolate. Purine deficiency states, while rare in humans, generally reflect a deficiency of folic acid. Compounds that inhibit formation of tetrahydrofolates and therefore block purine synthesis have been used in cancer chemotherapy. Inhibitory compounds and the reactions they inhibit include azaserine (reaction
, Figure 33–2), diazanorleucine (reaction
, Figure 33–2), 6-mercaptopurine (reactions
and
, Figure 33–3), and mycophenolic acid (reaction
, Figure 33–3).
“SALVAGE REACTIONS” CONVERT PURINES & THEIR NUCLEOSIDES TO MONONUCLEOTIDES
Conversion of purines, their ribonucleosides, and their deoxyribonucleosides to mononucleotides involves “salvage reactions” that require far less energy than de novo synthesis. The more important mechanism involves phosphoribosylation by PRPP (structure II, Figure 33–2) of a free purine (Pu) to form a purine 5′-mononucleotide (Pu-RP).
Phosphoryl transfer from ATP, catalyzed by adenosine-and hypoxanthine-phosphoribosyl transferases, converts adenine, hypoxanthine, and guanine to their mononucleotides (Figure 33–4).
FIGURE 33–4 Phosphoribosylation of adenine, hypoxanthine, and guanine to form AMP, IMP, and GMP, respectively.
A second salvage mechanism involves phosphoryl transfer from ATP to a purine ribonucleo side (Pu-R):
Phosphorylation of the purine nucleotides, catalyzed by adenosine kinase, converts adenosine and deoxyadenosine to AMP and dAMP. Similarly, deoxycytidine kinase phosphorylates deoxycytidine and 2′-deoxyguanosine, forming dCMP and dGMP.
Liver, the major site of purine nucleotide biosynthesis, provides purines and purine nucleosides for salvage and for utilization by tissues incapable of their biosynthesis. Human brain tissue has a low level of PRPP glutamyl amidotransferase (reaction , Figure 33–2) and hence depends in part on exogenous purines. Erythrocytes and polymorphonuclear leukocytes cannot synthesize 5-phosphoribosylamine (structure III, Figure 33–2) and therefore utilize exogenous purines to form nucleotides.
HEPATIC PURINE BIOSYNTHESIS IS STRINGENTLY REGULATED
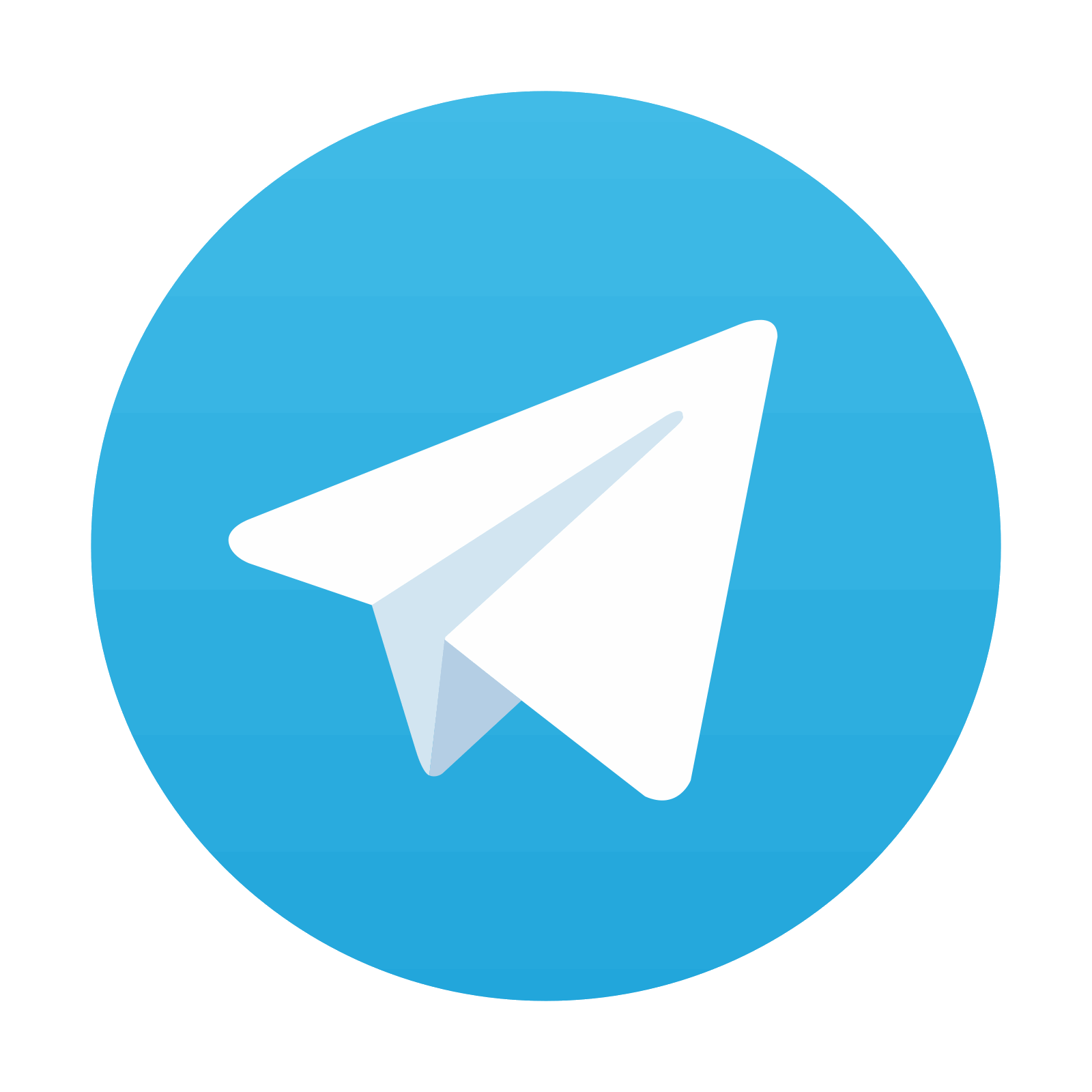
Stay updated, free articles. Join our Telegram channel
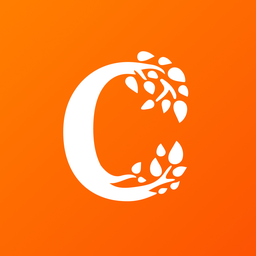
Full access? Get Clinical Tree
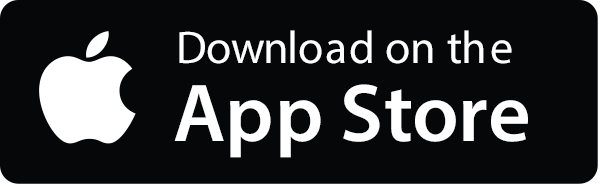
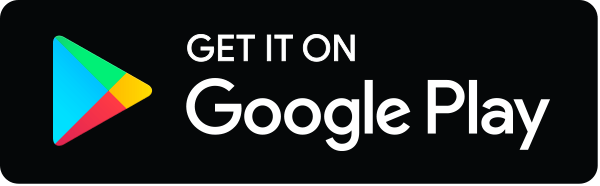