There are four major and a multitude of minor roles for lipids in living organisms. Major roles include serving as an energy source or fuel, structural components of membranes, lubricants (especially of the body surfaces), and signaling molecules. These four major functions require specific classes of lipids that differ in general structure. Each class contains numerous members with small but substantial structural differences. These lipid structures and their characteristics are discussed in Chapter 6. Lipids are important signaling molecules, both outside and inside cells. Sex hormones, adrenocortical hormones, and vitamin D are derived from cholesterol and play important extracellular signaling roles. Eicosanoids derived from arachidonic acid hydrolyzed from membrane phospholipids, and platelet-activating factor which is a phospholipid-like compound, are also important in extracellular signaling. Inside cells, fatty acids alone or compounds derived from them can be ligands for transcription factors, such as the peroxisome proliferator–activated receptors, to regulate transcription of specific genes. In addition, diacylglycerol and molecules derived from phospholipids and sphingolipids are involved in the transmission of signals from the plasma membrane to enzymes in the cytosol or other subcellular compartments and to proteins that regulate the expression of specific genes in the nucleus. In this chapter, synthesis and oxidation of fatty acids and acylglycerols as well as sphingolipids are discussed. Cholesterol and lipoprotein metabolism and transport are discussed in Chapter 17. Fat or triacylglycerol metabolism involves a series of hydrolysis and reesterification processes in the body. Free fatty acids are transported across membranes, but triacylglycerols are the major form of lipid in the large lipoprotein complexes in the circulation and in the lipid droplets present inside cells that store fat. Although the processes involved in fatty acid and triacylglycerol metabolism are described in detail in this chapter, a brief overview helps to place these pathways within the context of whole body metabolism. To illustrate these processes, the major aspects of lipid metabolism following intake of a carbohydrate- and fat-containing meal are shown in Figure 16-1, A. Dietary fat is digested in the small intestine and the products are converted back to triacylglycerols in the small intestinal absorptive cells where they are also incorporated into chylomicrons that are released into the lymphatic circulation and eventually the blood. When dietary carbohydrate is high, the liver synthesizes fatty acids de novo. These fatty acids as well as fatty acids returned to the liver by lipoprotein remnants are reesterified to triacylglycerol and incorporated into very-low-density lipoproteins (VLDLs) that are released by the liver into the venous circulation. The chylomicrons and VLDLs are acted upon by extracellular lipoprotein lipase in the capillaries of various tissues, in particular by adipose tissue lipoprotein lipase in the postprandial state. The fatty acids released from the triacylglycerol are taken up by adipose tissue where they are reesterified and stored as triacylglycerol. Reesterification can also occur in muscle for storage of fatty acids as intramyocellular triacylglycerol (IMTG). Fatty acids are also oxidized to CO2 and H2O by skeletal muscle; although resting muscle will be using mainly glucose as fuel in the postprandial period, working muscle will still use fatty acids as fuel. Glycerol (released from hydrolysis of chylomicron triacylglycerol) and the cholesterol-rich lipoprotein remnants (chylomicron remnants and the intermediate- and low-density lipoproteins, IDL and LDL, formed from VLDL) remain in the circulation and are subsequently taken up by the liver. The liver is able to convert glycerol to glycerol 3-phosphate and use it for esterification of fatty acids or as a glycolytic/gluconeogenic intermediate. During fasting or exercise, free or nonesterified fatty acids are released from the triacylglycerol stores in adipose tissue and are used as fuel by many tissues. Figure 16-1, B, illustrates the important role of adipose tissue as a source of free fatty acids. The triacylglycerol in lipid droplets in the adipocytes is hydrolyzed to fatty acids and glycerol, both of which are released into the venous circulation. These free fatty acids are subsequently oxidized by muscle and other tissues in a process known as β-oxidation. The glycerol is taken up by liver, as in the fed state. Although chylomicrons are not present in the fasting state, VLDL are still synthesized by the liver from lipids returned to the liver in the LDL and IDL and are available as a substrate for lipoprotein lipase in extrahepatic tissues, especially in the muscle. In prolonged starvation, the release of fatty acids is large and some of these will be used for ketone body synthesis in the liver. Ketone bodies in the circulation then serve as an additional lipid fuel for various tissues. The primary anatomical sites for synthesis of fatty acids are the liver and adipose tissue. In humans, the extent and the contribution of each of these tissues to de novo lipogenesis are still debated (Nye et al., 2008; Schutz, 2004). The lipogenic pathway may be suppressed by the high fat content of the modern diet (∼34% of total energy), and de novo lipogenesis may not contribute greatly to triacylglycerol biosynthesis in most individuals consuming western diets. However, low but regulated rates of lipogenesis still may be critical for overall control of metabolism in humans. The substrates and intermediates in pathways of lipid synthesis and oxidation are mainly thioesters of fatty acids and coenzyme A (CoA). As discussed in subsequent text, malonyl-CoA, the product of the acetyl-CoA carboxylase reaction, inhibits fatty acid transport into mitochondria, thereby controlling fatty acid metabolism. In addition, de novo lipogenesis may also contribute to glycemic control by diverting excess glucose to fat. Furthermore, in some physiological and pathophysiological conditions, de novo lipogenesis may play a quantitatively significant role. For example, developmental needs for lipid in the fetus may be met by de novo lipogenesis, the rate of which is extremely high in premature infants. De novo lipogenesis also contributes significantly to hypertriglyceridemia and hepatic steatosis. Some of the metabolic abnormalities in untreated type 1 diabetes mellitus arise from the impaired fatty acid synthesis caused by low insulin levels. Another calorically important site of fat synthesis is the lactating mammary gland, in which medium-chain fatty acids are synthesized and esterified to glycerol for milk fat. Branched-chain fatty acids for conditioning body surfaces are synthesized in sebaceous and other more specialized glands. The substrate for fatty acid synthesis, acetyl-CoA, is formed from pyruvate in the mitochondria. The inner mitochondrial membrane is impermeable to acetyl-CoA and does not contain a carrier to transport the acetyl-CoA into the cytosol. When production of acetyl-CoA from pyruvate is high, the rate of formation of citrate catalyzed by citrate synthase in the citric acid cycle also is elevated, resulting in the accumulation of intramitochondrial citrate. Under these conditions, citrate can be translocated to the cytosol in exchange for a dicarboxylate anion, probably malate, by the tricarboxylate anion carrier in the inner mitochondrial membrane, as shown in Figure 16-2. Citrate, therefore, serves as the intermediary for the transfer of acetyl-CoA from mitochondria to cytosol. As described in subsequent text of this chapter, citrate as a feed-forward activator of acetyl-CoA carboxylase plays a key role in regulating fatty acid synthesis. In the cytosol, therefore, fatty acid synthesis actually starts by cleavage of citrate back to acetyl-CoA. In the first step, the biotin, which is bound to a specific lysine residue of the enzyme, is carboxylated with the energy furnished by the hydrolysis of ATP (biotin carboxylation). In the second step (transcarboxylation), the activated, biotin-bound carboxyl group is transferred to acetyl-CoA, regenerating enzyme-bound biotin and synthesizing malonyl-CoA. Both of these reactions are catalyzed by a single polypeptide chain that also contains a domain for the covalently linked biotin. In biotin deficiency, the acetyl-CoA carboxylase reaction is impaired, and thus fatty acid synthesis from acetyl-CoA is decreased. (See Chapter 26 for further discussion of biotin-dependent carboxylation.) Acetyl-CoA carboxylase is regulated by an array of control mechanisms, thereby permitting the rate of fatty acid synthesis to fluctuate in response to physiological and developmental conditions (Sul and Smith, 2008; Wakil and Abu-Elheiga, 2009). At the same time, malonyl-CoA, the product of acetyl-CoA carboxylase, is a potent inhibitor of carnitine palmitoyltransferase 1 (CPT1), an enzyme that controls transport of long-chain fatty acids into mitochondria for oxidation (as discussed in subsequent text). Therefore acetyl-CoA carboxylase reciprocally regulates fatty acid synthesis and oxidation. There are two isoforms of acetyl-CoA carboxylase: ACC1 is for acetyl-CoA synthesis in lipogenic tissues (i.e., liver and adipose tissue), whereas ACC2 present in muscle and other tissues is for formation of malonyl-CoA for regulating fatty acid oxidation. The activity of acetyl-CoA carboxylase is stimulated by citrate, an allosteric activator, and inhibited by long-chain acyl-CoA, an allosteric inhibitor. In the fed state, production of cytosolic citrate is increased and the concentration of long-chain acyl-CoA is low, resulting in activation of acetyl-CoA carboxylase. Conversely, during starvation, the long-chain acyl-CoA level increases and the cytosolic citrate level decreases, resulting in inhibition of fatty acid synthesis. Activation of lipolysis in the adipose tissue gives rise to the increase in long-chain acyl-CoA concentration, and the lack of excess anaphlerotic substrate (e.g., pyruvate, oxaloacetate) for citrate formation in the mitochondrial citric acid cycle gives rise to the lack of cytosolic citrate. These allosteric mechanisms thus represent examples of feed-forward (citrate) and feedback (long-chain acyl-CoA) regulation of acetyl-CoA carboxylase as shown in Figure 16-3. Acetyl-CoA carboxylase also is regulated by covalent modification (Brownsey et al., 2006; Saggerson, 2008), as shown in Figure 16-3. Up to seven serine residues in acetyl-CoA carboxylase can be phosphorylated. The phosphorylated enzyme is less active, less sensitive to the stimulatory effects of citrate, and more sensitive to the inhibitory action of long-chain acyl-CoA. A number of different protein kinases catalyze phosphorylation of acetyl-CoA carboxylase and do so at different specific serine residues on the enzyme. 5′-AMP–activated protein kinase (AMPK) is the physiologically important kinase that phosphorylates acetyl-CoA carboxylase. When the cellular energy state is low, with increased intracellular AMP levels, AMPK is allosterically activated by AMP. AMPK is also activated by phosphorylation by upstream kinases such as LKB1, and phosphorylation of AMPK is promoted by AMP (Steinberg and Kemp, 2009). Activated AMPK in turn phosphorylates acetyl-CoA carboxylase resulting in a decrease in its activity. In liver, glucagon is an important effector increasing production of 3′-5′-cyclic adenosine monophosphate (cAMP). Catecholamines can also increase production of cAMP. An increase in cAMP in turn activates protein kinase A (see Figure 16-3). There may be a potential physiological role for protein kinase A in the hormone-mediated inactivation of acetyl-CoA carboxylase. However, regulation of acetyl-CoA carboxylase activity by protein kinase A–mediated phosphorylation is somewhat smaller in magnitude than that by AMPK-mediated phosphorylation. It is also possible that protein kinase A regulation of acetyl-CoA carboxylase may not occur via direct phosphorylation of this enzyme. In addition to regulation by allosteric and phosphorylation–dephosphorylation mechanisms, acetyl-CoA carboxylase also is regulated by changes in the number of molecules present in the cell. The concentration of the enzyme is low in the liver of starved animals and high in the liver of fed animals, especially if the diet is high in carbohydrate. The acetyl-CoA carboxylase protein concentration in cells is controlled primarily by changes in the rate of transcription of the acetyl-CoA carboxylase gene. In this regard, in addition to acetyl-CoA carboxylase, transcription of many of the enzymes involved in fatty acid synthesis are coordinately regulated. These transcriptionally regulated enzymes include ATP-citrate lyase, which hydrolyzes citrate to provide the cytosolic acetyl-CoA that is used as a substrate for acetyl-CoA carboxylase and fatty acid synthase, and malic enzyme and some of the enzymes in the pentose phosphate pathway that generate the NADPH required for fatty acid synthase. Transcription of these enzymes is high in the fed state (especially if the diet is high in carbohydrate) and low in the fasting state. Fat, especially polyunsaturated fatty acids in the diet, decreases transcription. Changes in circulating insulin and glucagon, as well as changes in glucose levels, participate in the transcriptional regulation of lipogenic enzymes, including acetyl-CoA carboxylase. Insulin and glucose increase, whereas glucagon and catecholamines (via cAMP) decrease, the rate of transcription of acetyl-CoA carboxylase. When glucose levels are elevated in the fed state, insulin signaling via the insulin/phosphatidylinositol 3-kinase/Akt pathway results in activation of sterol regulatory element binding protein (SREBP) 1c, a basic helix-loop-helix transcription factor that resides at the ER but, upon cleavage, becomes a mature transcription factor and translocates to the nucleus to activate lipogenic gene transcription in the fed state. SREBP1c is expressed predominantly in the lipogenic tissues and SREBP1c gene transcription is itself suppressed in the fasted state but induced by high carbohydrate feeding. Liver X receptor (LXR), a ligand activated nuclear hormone receptor, activates transcription of SREBP1c as well as lipogenic enzymes in the fed state. LXR also increases transcription of another basic helix-loop-helix transcription factor, carbohydrate response element binding protein (ChREBP), which mediates transcriptional activation of lipogenic enzymes by glucose (Wong et al., 2009, Wong and Sul, 2010). The function of SREBP is discussed in more detail in Chapter 17. The second and final committed step in fatty acid synthesis is catalyzed by a multifunctional polypeptide, fatty acid synthase, which catalyzes all the reactions shown in Figure 16-4 and which contains an acyl carrier protein (ACP) domain with a 4′-phosphopantetheine prosthetic group. The substrates for the synthesis of one palmitate molecule by fatty acid synthase are 7 molecules of malonyl-CoA, 1 of acetyl-CoA, 14 of NADPH, and 14 of H+. The products are 1 molecule of palmitate, 8 of CoA, 7 of CO2, 14 of NADP, and 6 of H2O. The reaction is complex in two ways. First, the fatty acid is built up by the serial addition of two-carbon fragments to a growing chain. Second, after each addition, the added carbons are reduced, dehydrated, and reduced again. The process then repeats itself—condensation, reduction, dehydration, and reduction—until a 16-carbon fatty acid (palmitate) has been formed and is released from the enzyme. acetyl-CoA to a serine residue in the acyltransferase domain of the enzyme. The acetyl group is then transferred from the serine residue in the acyltransferase domain to the 4′-phosphopantetheine if the sulfhydryl of the covalently linked 4′-phosphopantetheine group in the acyl carrier protein domain of the enzyme is free. The 4′-phosphopantetheine group of the ACP domain of fatty acid synthase is described in Chapter 26. The β-ketoacyl synthase (the “condensing enzyme”) activity of fatty acid synthase then catalyzes transfer of the acetyl group to a cysteine residue at the active site of the condensation reaction. The serine residue in the acyl transferase domain is now free to accept a malonyl group. The malonyl group is then transferred to the sulfhydryl group of the phosphopantetheine side arm (see Figure 16-4), which has been freed of its acetyl group, and the enzyme is poised to carry out the first condensation reaction. The phosphopantetheine side arm in the ACP domain of fatty acid synthase is long and flexible, so that its attached acetoacetyl group can interact sequentially with the active sites of the β-ketoacyl reductase, β-hydroxyacyl dehydratase, and enoyl reductase domains to form a 4-carbon saturated fatty acyl group. At the end of this reaction sequence, the butyryl moiety remains attached to the phosphopantetheinyl moiety, but it is then transferred to the cysteine residue in the active site of the β-ketoacyl synthase domain. This leaves the sulfhydryl of the phosphopantetheinyl moiety free to receive a new malonyl-CoA moiety from the serine residue in the acyltransferase domain. The next cycle of condensation, reduction, dehydration, and reduction then forms a 6-carbon saturated fatty-acyl group (hexanoyl) attached to the phosphopantetheine side arm. The hexanoyl group is transferred to the active site cysteine residue of the β-ketoacyl synthase domain, and another malonyl group is condensed, reduced, dehydrated, and reduced. After seven cycles, the growing acyl chain reaches 16 carbons, and a thioesterase activity in the thioesterase domain of fatty acid synthase cleaves the free fatty acid from the enzyme (see Figure 16-4). As mentioned previously, all of the reactions required for fatty acid synthesis from acetyl-CoA and malonyl-CoA are catalyzed by a single polypeptide (i.e., fatty acid synthase) that contains all activities in separate domains. The multifunctional fatty acid synthase can provide a greater efficiency by channeling intermediates from one active site to the next rather than relying on diffusion of intermediates between separate enzymes. In addition, the amounts of each enzyme can be regulated simultaneously by controlling expression of a single gene. Mammalian fatty acid synthase is synthesized as an inactive monomer of about 270 kDa (Figure 16-5). The active enzyme is an intertwined head-to-head homodimer of two fatty acid synthase monomers that has an X shape, with each of the two lateral clefts of the X defining a reaction chamber (Maier et al., 2008). One complete set of domains for progressive elongation of the fatty acid chain is present in each of the lateral clefts. Similar to acetyl CoA carboxylase, fatty acid synthase activity is low in the fasted state but increases drastically in the fed state, especially when the diet is rich in carbohydrate. However, neither allosteric nor phosphorylation–dephosphorylation mechanisms appear to contribute significantly to these changes in fatty acid synthase activity. The major regulation is at the level of protein concentration as a consequence of changes in gene transcription by the previously described mechanism involving several transcription factors, including SREBP-1c (Wong and Sul, 2010). Fatty acids can be elongated in two-carbon steps. Elongation of fatty acids occurs in the endoplasmic reticulum (ER), mitochondria, and peroxisomes. In general, the enzyme systems for elongation are not well understood, but the ER elongation system is the most active and most studied. The ER elongation system uses both saturated and unsaturated fatty acyl-CoAs as substrates. The individual reactions are analogous to those catalyzed by fatty acid synthase, except that long-chain acyl-CoAs are used as primers rather than acetyl-CoA, and different gene products catalyze the individual reactions. The sequence of reactions is condensation, reduction, dehydration, and reduction. In the ER, malonyl-CoA is the elongating group, and NADPH is the electron donor. The initial rate-controlling step of a condensation reaction is referred to as elongation of very-long-chain fatty acids (ELOVL), and there are at least seven ELOVL enzymes that prefer either saturated and monounsaturated fatty acids or polyunsaturated fatty acids (Guillou et al., 2010). The mitochondrial elongation system appears to use acetyl-CoA as the elongating unit and NADH or NADH plus NADPH as electron donors. The mitochondrial elongation system is not a simple reversal of fatty acid oxidation involving two-carbon units (β-oxidation) because flavoenzymes are not involved in catalysis for either the first or the second reduction in each cycle. Fatty acid elongation in peroxisomes is even less understood but may produce very-long-chain fatty acids of 24 to 36 carbons in length. Monounsaturated fatty acids are formed by direct oxidative desaturation of preformed long-chain saturated fatty acids in reactions catalyzed by a complex of enzymes located in the ER. The first double bond introduced into a saturated acyl chain is generally in the Δ9 position. The Δ9 desaturase complex (also called stearoyl-CoA desaturase, SCD) uses saturated fatty acids with 14 to 18 carbons; stearate is the most active. This complex, which is sometimes called a mixed function oxidase, uses two electrons and two protons donated by NADH (+ H+), two electrons and two protons from the fatty acid, and oxygen (as an electron acceptor) to generate the unsaturated fatty acid and two water molecules, as shown in Figure 16-6. The substrates and products are the acyl-CoA derivatives of the fatty acid. Electrons donated by NADH are passed via FADH2 to the heme iron of cytochrome b5, reducing it to the ferrous form, in a reaction catalyzed by NADH:cytochrome b5 reductase. Cytochrome b5 then donates two electrons to the nonheme iron of the desaturase component, reducing it to the ferrous form. This form then interacts with molecular oxygen and the fatty acyl-CoA to form water and the unsaturated fatty acyl-CoA. The desaturase activity regulates the overall reaction. Multiple forms of Δ9 desaturase are present with differing tissue distributions and regulation. The activity of SCD1, the isozyme present mostly in liver and adipose tissue, is controlled by diet and hormones in much the same manner as are the activities of the lipogenic enzymes, mainly by regulation of enzyme concentration. Multiple desaturation and elongation reactions, plus a retroconversion step by peroxisomal β-oxidation, are involved in synthesis of docosahexaenoate (22:6, Δ4, Δ7, Δ10, Δ13, Δ16, Δ19) from α-linolenate. (See Chapters 6 and 18 for further discussion of essential fatty acids and fatty acid nomenclature.) Fatty acids to be used for synthesis of triacylglycerol comprise fatty acids from de novo synthesis and those from hydrolysis of triacylglycerols in the cell, as well as fatty acids taken up from the circulation. The fatty acids taken up from the circulation may be free fatty acids (mainly released by lipolysis and associated with plasma albumin) or fatty acids generated locally from circulating lipoproteins (by the action of lipoprotein lipases localized on the endothelium of the capillaries within the tissue). These fatty acids from the plasma are taken up by the cell either by passive diffusion or by plasma membrane fatty acid transporters, such as fatty acid translocase (FAT/CD36), fatty acid transport proteins (FATPs), or plasma membrane fatty acid binding protein (FABPpm) (Glatz et al., 2010; Su and Abumrad, 2008). Because of their hydrophobic properties, once inside the cell, long-chain fatty acids are bound to fatty acid binding proteins (FABPs) to be transferred from membrane to membrane within the cell (Storch and Corsico, 2008). The glycerol 3-phosphate reaction pathway for the synthesis of triacylglycerols starts with fatty acyl-CoA and glycerol 3-phosphate, as shown in Figure 16-7. The acyl group of a fatty acyl-CoA is transferred to the sn-1 position of glycerol 3-phosphate in the reaction catalyzed by glycerol 3-phosphate acyltransferase. This is the first committed step in glycerolipid biosynthesis.
Metabolism of Fatty Acids, Acylglycerols, and Sphingolipids
Biological Roles for Lipids
Overview of Fatty Acid and Triacylglycerol Metabolism
Synthesis of Palmitate from Acetyl-CoA
Transfer of Acetyl-CoA from Inside the Mitochondria to the Cytosol
Conversion of Acetyl-CoA to Malonyl-CoA
Synthesis of Palmitate from Malonyl-CoA, Acetyl-CoA, and NADPH by Fatty Acid Synthase
Synthesis of Fatty Acids other than Palmitate
Elongation of Fatty Acids
Desaturation of Fatty Acids
Synthesis of Triacylglycerol
Sources of Fatty Acids for Triacylglycerol Synthesis
Esterificaton of Fatty Acids
Stay updated, free articles. Join our Telegram channel
Full access? Get Clinical Tree
Metabolism of Fatty Acids, Acylglycerols, and Sphingolipids
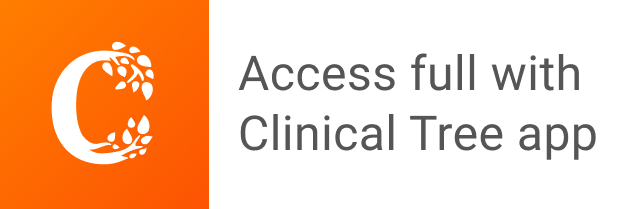