40
Membranes: Structure & Function
Robert K. Murray, MD, PhD & Daryl K. Granner, MD
OBJECTIVES
After studying this chapter, you should be able to:
Know that biological membranes are mainly composed of a lipid bilayer and associated proteins and glycoproteins. The major lipids are phospholipids, cholesterol, and glycosphingolipids.
Appreciate that membranes are asymmetric, dynamic structures containing a mixture of integral and peripheral proteins.
Know the fluid mosaic model of membrane structure and that it is widely accepted, with lipid rafts, caveolae, and tight junctions being specialized features.
Understand the concepts of passive diffusion, facilitated diffusion, active transport, endocytosis, and exocytosis.
Recognize that transporters, ion channels, the Na+-K+-ATPase, receptors, and gap junctions are important participants in membrane function.
Know that a variety of disorders result from abnormalities of membrane structure and function, including familial hypercholesterolemia, cystic fibrosis, hereditary spherocytosis, and many others.
BIOMEDICAL IMPORTANCE
Membranes are highly fluid, dynamic structures consisting of a lipid bilayer and associated proteins. Plasma membranes form closed compartments around the cytoplasm to define cell boundaries. The plasma membrane has selective permeabilities and acts as a barrier, thereby maintaining differences in composition between the inside and outside of the cell. The selective permeabilities for substrates and ions are provided mainly by specific proteins named transporters and ion channels. The plasma membrane also exchanges material with the extracellular environment by exocytosis and endocytosis, and there are special areas of membrane structure—gap junctions— through which adjacent cells exchange material. In addition, the plasma membrane plays key roles in cell-cell interactions and in transmembrane signaling.
Membranes also form specialized compartments within the cell. Such intracellular membranes help shape many of the morphologically distinguishable structures (organelles), eg, mitochondria, ER, Golgi, secretory granules, lysosomes, and the nucleus. Membranes localize enzymes, function as integral elements in excitation-response coupling, and provide sites of energy transduction, such as in photosynthesis and oxidative phosphorylation.
Changes in membrane components can affect water balance and ion flux, and therefore many processes within the cell. Specific deficiencies or alterations of certain membrane components (eg, caused by mutations genes encoding membrane proteins) lead to a variety of diseases (see Table 40-7). In short, normal cellular function depends on normal membranes.
MAINTENANCE OF A NORMAL INTRA- & EXTRACELLULAR ENVIRONMENT IS FUNDAMENTAL TO LIFE
Life originated in an aqueous environment; enzyme reactions, cellular and subcellular processes, and so forth have therefore evolved to work in this milieu, encapsulated within a cell.
The Body’s Internal Water Is Compartmentalized
Water makes up about 60% of the lean body mass of the human body and is distributed in two large compartments.
Intracellular Fluid (ICF)
This compartment constitutes two-thirds of total body water and provides a specialized environment for the cell (1) to make, store, and utilize energy; (2) to repair itself; (3) to replicate; and (4) to perform cell-specific functions.
Extracellular Fluid (ECF)
This compartment contains about one-third of total body water and is distributed between the plasma and interstitial compartments. The extracellular fluid is a delivery system. It brings to the cells nutrients (eg, glucose, fatty acids, and amino acids), oxygen, various ions and trace minerals, and a variety of regulatory molecules (hormones) that coordinate the functions of widely separated cells. Extracellular fluid removes CO2, waste products, and toxic or detoxified materials from the immediate cellular environment.
The Ionic Compositions of Intracellular & Extracellular Fluids Differ Greatly
As illustrated in Table 40-1, the internal environment is rich in K+ and Mg2+, and phosphate is its major inorganic anion. The cytosol of cells contains a high concentration of protein that acts as a major intracellular buffer. Extracellular fluid is characterized by high Na+ and Ca2+ content, and Cl– is the major anion. Why are there such differences? It is thought that the primordial sea in which life originated was rich in K+ and Mg2+. It, therefore, follows that enzyme reactions and other biologic processes evolved to function best in that environment—hence, the high concentration of these ions within cells. Vast changes would have been required for evolution of a completely new set of biochemical and physiologic machinery; instead, as it happened, cells developed barriers—membranes with associated “pumps” such as the Na+-K+-ATPase (see below)—to maintain the internal microenvironment.
TABLE 40–1 Comparison of the Mean Concentrations of Various Molecules Outside and Inside a Mammalian Cell
MEMBRANES ARE COMPLEX STRUCTURES COMPOSED OF LIPIDS, PROTEINS, & CARBOHYDRATE-CONTAINING MOLECULES
We shall mainly discuss the membranes present in eukaryotic cells, although many of the principles described also apply to the membranes of prokaryotes. The various cellular membranes have different compositions, as reflected in the ratio of protein to lipid (Figure 40–1). This is not surprising, given their divergent functions. Membranes are sheet-like enclosed structures consisting of an asymmetric lipid bilayer with distinct inner and outer surfaces. These sheet-like structures are non-covalent assemblies that form spontaneously in water due to the amphipathic nature of lipids. Many different proteins are located in membranes, where they carry out specific functions.
FIGURE 40–1 Ratio of protein to lipid in different membranes. Proteins equal or exceed the quantity of lipid in nearly all membranes. The outstanding exception is myelin, an electrical insulator found on many nerve fibers.
The Major Lipids in Mammalian Membranes Are Phospholipids, Glycosphingolipids & Cholesterol
Phospholipids
Of the two major phospholipid classes present in membranes, phosphoglycerides are the more common and consist of a glycerol backbone to which are attached two fatty acids in ester linkages and a phosphorylated alcohol (Figure 40–2). The fatty acid constituents are usually even-numbered carbon molecules, most commonly containing 16 or 18 carbons. They are unbranched and can be saturated or unsaturated with one or more cis double bonds. The simplest phosphoglyceride is phosphatidic acid, which is 1,2-diacylglycerol 3-phosphate, a key intermediate in the formation of other phosphoglycerides (Chapter 24). In most phosphoglycerides present in membranes, the 3-phosphate is esterified to an alcohol such as choline, ethanolamine, glycerol, inositol or serine (Chapter 15). Phosphatidylcholine is generally the major phosphoglyceride by mass in the membranes of human cells.
FIGURE 40–2 A phosphoglyceride showing the fatty acids (R1 and R2), glycerol, and a phosphorylated alcohol component. Saturated fatty acids are usually attached to carbon 1 of glycerol, and unsaturated fatty acids to carbon 2. In phosphatidic acid, R3 is hydrogen.
The second major class of phospholipids comprises sphingomyelin (Figure 15–13), which contains a sphingosine backbone rather than glycerol. A fatty acid is attached by an amide linkage to the amino group of sphingosine, forming ceramide. When the primary hydroxyl group of sphingosine is esterified to phosphorylcholine, sphingomyelin is formed. As the name implies, sphingomyelin is prominent in myelin sheaths.
Glycosphingolipids
The glycosphingolipids (GSLs) are sugar-containing lipids built on a backbone of ceramide; they include galactosyl- and glucosylceramide (cerebrosides) and the gangliosides. Their structures are described in Chapter 15. They are mainly located in the plasma membranes of cells, displaying their sugar components to the exterior of the cell.
Sterols
The most common sterol in the membranes of animal cells is cholesterol (Chapter 15), which resides mainly in their plasma membranes, but can also be found in lesser quantities in mitochondria, Golgi complexes, and nuclear membranes. Cholesterol intercalates among the phospholipids of the membrane, with its hydroxyl group at the aqueous interface and the remainder of the molecule within the leaflet. Its effect on the fluidity of membranes will be discussed subsequently. From a nutritional viewpoint, it is important to know that cholesterol is not present in plants.
Lipids can be separated from one another and quantitated by techniques such as column, thin-layer, and gas-liquid chromatography and their structures can be established by mass spectrometry and other techniques.
Membrane Lipids Are Amphipathic
All major lipids in membranes contain both hydrophobic and hydrophilic regions and are therefore termed amphipathic. If the hydrophobic region were separated from the rest of the molecule, it would be insoluble in water but soluble in oil. Conversely, if the hydrophilic region were separated from the rest of the molecule, it would be insoluble in oil but soluble in water. The amphipathic nature of a phospholipid is represented in Figure 40–3 and also Figure 15–24. Thus, the polar head groups of the phospholipids and the hydroxyl group of cholesterol interface with the aqueous environment; a similar situation applies to the sugar moieties of the GSLs (see below).
FIGURE 40–3 Diagrammatic representation of a phospholipid or other membrane lipid. The polar head group is hydrophilic, and the hydrocarbon tails are hydrophobic or lipophilic. The fatty acids in the tails are saturated (S) or unsaturated (U); the former are usually attached to carbon 1 of glycerol and the latter to carbon 2 (see Figure 40–2). Note the kink in the tail of the unsaturated fatty acid (U), which is important in conferring increased membrane fluidity.
Saturated fatty acids have straight tails, whereas unsaturated fatty acids, which generally exist in the cis form in membranes, make kinked tails (Figure 40–3). As more kinks are inserted in the tails, the lipids become less tightly packed and the membrane more fluid. The problem caused by the presence of trans fatty acids in membrane lipids is described in Chapter 15.
Detergents are amphipathic molecules that are important in biochemistry as well as in the household. The molecular structure of a detergent is not unlike that of a phospholipid. Certain detergents are widely used to solubilize membrane proteins and in their purification. The hydrophobic end of the detergent binds to hydrophobic regions of the proteins, displacing most of their bound lipids. The polar end of the detergent is free, bringing the proteins into solution as detergent-protein complexes, usually also containing some residual lipids.
Membrane Lipids Form Bilayers
The amphipathic character of phospholipids suggests that the two regions of the molecule have incompatible solubilities; however, in a solvent such as water, phospholipids organize themselves into a form that thermodynamically serves the solubility requirements of both regions. A micelle (Figure 40–4 and Figure 15–24) is such a structure; the hydrophobic regions are shielded from water, while the hydrophilic polar groups are immersed in the aqueous environment. However, micelles are usually relatively small in size (eg, ~200 nm) and thus are limited in their potential to form membranes. Detergents commonly form micelles.
FIGURE 40–4 Diagrammatic cross-section of a micelle. The polar head groups are bathed in water, whereas the hydrophobic hydrocarbon tails are surrounded by other hydrocarbons and thereby protected from water. Micelles are relatively small (compared with lipid bilayers) spherical structures.
As was recognized in 1925 by Gorter and Grendel, a bimolecular layer, or lipid bilayer, can also satisfy the thermodynamic requirements of amphipathic molecules in an aqueous environment. Bilayers are the key structures in biological membranes. A bilayer exists as a sheet in which the hydrophobic regions of the phospholipids are sequestered from the aqueous environment, while the hydrophilic regions are exposed to water (Figure 40–5 and Figure 15–24). The ends or edges of the bilayer sheet can be eliminated by folding the sheet back upon itself to form an enclosed vesicle with no edges. The closed bilayer provides one of the most essential properties of membranes. It is impermeable to most water-soluble molecules since they would be insoluble in the hydrophobic core of the bilayer.
FIGURE 40–5 Diagram of a section of a bilayer membrane formed from phospholipid molecules. The unsaturated fatty acid tails are kinked and lead to more spacing between the polar head groups, hence to more room for movement. This in turn results in increased membrane fluidity. (Slightly modified and reproduced, with permission, from Stryer L: Biochemistry, 2nd ed. Freeman, 1981. Copyright ©1981 by W H. Freeman and Company.)
Lipid bilayers are formed by self-assembly, driven by the hydrophobic effect (Chapter 2). When lipid molecules come together in a bilayer, the entropy of the surrounding solvent molecules increases due to the release of immobilized water.
Two questions arise from consideration of the above. First, how many biologic materials are lipid-soluble and can therefore readily enter the cell? Gases such as oxygen, CO2, and nitrogen—small molecules with little interaction with solvents—readily diffuse through the hydrophobic regions of the membrane. The permeability coefficients of several ions and of a number of other molecules in a lipid bilayer are shown in Figure 40–6. The three electrolytes shown (Na+, K+, and Cl–) cross the bilayer much more slowly than water. In general, the permeability coefficients of small molecules in a lipid bilayer correlate with their solubilities in nonpolar solvents. For instance, steroids more readily traverse the lipid bilayer compared with electrolytes. The high permeability coefficient of water itself is surprising, but is partly explained by its small size and relative lack of charge. Many drugs are hydrophobic and can readily cross membranes and enter cells.
FIGURE 40–6 Permeability coefficients of water, some ions, and other small molecules in lipid bilayer membranes. The permeability coefficient is a measure of the ability of a molecule to diffuse across a permeability barrier. Molecules that move rapidly through a given membrane are said to have a high permeability coefficient. (Slightly modified and reproduced, with permission, from Stryer L: Biochemistry, 2nd ed. Freeman, 1981. Copyright © 1981)
The second question concerns molecules that are not lipid-soluble: How are the transmembrane concentration gradients for nonlipid-soluble molecules maintained? The answer is that membranes contain proteins, many of which span the lipid bilayer. Such proteins form channels for the movement of ions and small molecules or serve as transporters for molecules that otherwise could not pass the bilayer. These structures are described below.
Membrane Proteins Are Associated with the Lipid Bilayer
Membrane phospholipids act as a solvent for membrane proteins, creating an environment in which the latter can function. As described in Chapter 5, the α-helical structure of proteins minimizes the hydrophilic character of the peptide bonds themselves. Thus, proteins can be amphipathic and form an integral part of the membrane by having hydrophilic regions protruding at the inside and outside faces of the membrane but connected by a hydrophobic region traversing the hydrophobic core of the bilayer. In fact, those portions of membrane proteins that traverse membranes do contain substantial numbers of hydrophobic amino acids and almost invariably have a high α-helical content. For many membranes, a stretch of ~20 amino acids in an α-helix will span the bilayer.
It is possible to calculate whether a particular sequence of amino acids present in a protein is consistent with a transmembrane location. This can be done by consulting a Table that lists the hydrophobicities of each of the 20 common amino acids and the free energy values for their transfer from the interior of a membrane to water. Hydrophobic amino acids have positive values; polar amino acids have negative values. The total free energy values for transferring successive sequences of 20 amino acids in the protein are plotted, yielding a so-called hydropathy plot. Values of over 20 kcal mol-1 are consistent with—but do not prove—the interpretation that the hydrophobic sequence is a transmembrane segment.
Another aspect of the interaction of lipids and proteins is that some proteins are anchored to one leaflet of the bilayer by covalent linkages to certain lipids. Palmitate and myristate are fatty acids involved in such linkages to specific cytosolic proteins. A number of cell surface proteins (see Chapter 47) are linked to the plasma membrane via glycophosphatidylinositol (GPI) structures.
Different Membranes Have Different Protein Compositions
The number of different proteins in a membrane varies from less than a dozen in the sarcoplasmic reticulum of muscle cells to over 100 in plasma membranes. Membrane proteins can be separated from one another using sodium dodecyl sulfate polyacrylamide gel electrophoresis (SDS-PAGE), a technique that separates proteins based on their molecular mass. Using standard proteins of known molecular mass as a comparison, one can estimate the approximate molecular mass of an unknown protein via SDS-PAGE. SDS is a powerful detergent that disrupts protein-lipid interactions and thereby solubilizes membrane proteins. SDS also disrupts protein-protein interactions and unfolds or denatures proteins. In the absence of SDS, few membrane proteins would remain soluble.
Proteins are the major functional molecules of membranes and consist of enzymes, pumps and transporters, channels, structural components, antigens (eg, for histocompatibility), and receptors for various molecules. Because every type of membrane possesses a different complement of proteins, there is no such thing as a typical membrane structure. The enzymatic properties of several different membranes are shown in Table 40-2.
TABLE 40–2 Enzymatic Markers of Different Membranes1
Membranes Are Dynamic Structures
Membranes and their components are dynamic structures. The lipids and proteins in membranes undergo turnover, just as they do in other compartments of the cell. Different lipids have different turnover rates, and the turnover rates of individual species of membrane proteins may vary widely. The membrane itself can turn over even more rapidly than any of its constituents. This is discussed in more detail in the section on endocytosis.
Another indicator of the dynamic nature of membranes is that a variety of studies have shown that lipids and certain proteins exhibit lateral diffusion in the plane of their membranes. Some proteins do not exhibit lateral diffusion because they are anchored to the underlying actin cytoskeleton. In contrast, the transverse movement of lipids across the membrane (flip-flop) is extremely slow (see below) and does not occur at all in the case of membrane proteins.
Membranes Are Asymmetric Structures
Proteins have unique orientations in membranes, making the outside surfaces different from the inside surfaces. An inside-outside asymmetry is also provided by the external location of the carbohydrates attached to membrane proteins. In addition, specific proteins are located exclusively on the outsides or insides of membranes.
There are also regional heterogeneities in membranes. Some, such as occur at the villous borders of mucosal cells, are almost macroscopically visible. Others, such as those at gap junctions, tight junctions, and synapses, occupy much smaller regions of the membrane and generate correspondingly smaller local asymmetries.
There is also inside-outside asymmetry of the phospholipids. The choline-containing phospholipids (phosphatidylcholine and sphingomyelin) are located mainly in the outer leaflet; the aminophospholipids (phosphatidylserine and phosphatidylethanolamine) are preferentially located in the inner leaflet. Obviously, if this asymmetry is to exist at all, there must be limited transverse mobility (flip-flop) of the membrane phospholipids. In fact, phospholipids in synthetic bilayers exhibit an extraordinarily slow rate of flip-flop; the half-life of the asymmetry can be measured in several weeks.
The mechanisms involved in the establishment of lipid asymmetry are not well understood. The enzymes involved in the synthesis of phospholipids are located on the cytoplasmic side of microsomal membrane vesicles. Translocases (flip-pases) exist that transfer certain phospholipids (eg, phosphatidylcholine) from the inner to the outer leaflet. Specific proteins that preferentially bind individual phospholipids also appear to be present in the two leaflets, contributing to the asymmetric distribution of these lipid molecules. In addition, phospholipid exchange proteins recognize specific phospholipids and transfer them from one membrane (eg, the endoplasmic reticulum [ER]) to others (eg, mitochondrial and peroxisomal). A related issue is how lipids enter membranes. This has not been studied as intensively as the topic of how proteins enter membranes (see Chapter 46) and knowledge is still relatively meager. Many membrane lipids are synthesized in the ER. At least three pathways have been recognized. (1) Transport from the ER in vesicles, which then transfer the contained lipids to the recipient membrane. (2) Entry via direct contact of one membrane (eg, the ER) with another, facilitated by specific proteins. (3) Transport via the phospholipid exchange proteins (also known as lipid transfer proteins) mentioned above. This only exchanges lipids, but does not cause net transfer.
There is further asymmetry with regard to glycosphingolipids and glycoproteins; the sugar moieties of these molecules all protrude outward from the plasma membrane and are absent from its inner face. Thus, cells are “sugar coated”.
Membranes Contain Integral & Peripheral Proteins
It is useful to classify membrane proteins into two types: integral and peripheral (Figure 40–7). Most membrane proteins fall into the integral class, meaning that they interact extensively with the phospholipids and require the use of detergents for their solubilization. Also, they generally span the bilayer as a bundle of α-helical transmembrane segments. Integral proteins are usually globular and are themselves amphipathic. They consist of two hydrophilic ends separated by an intervening hydrophobic region that traverses the hydrophobic core of the bilayer. As the structures of integral membrane proteins were being elucidated, it became apparent that certain ones (eg, transporter molecules, ion channels, various receptors, and G proteins) span the bilayer many times (see Figure 46–7), whereas other simple membrane proteins (eg, glycophorin A) span the membrane only once, Integral proteins are asymmetrically distributed across the membrane bilayer. This asymmetric orientation is conferred at the time of their insertion in the lipid bilayer during biosynthesis in the ER. The molecular mechanisms involved in insertion of proteins into membranes and the topic of membrane assembly are discussed in Chapter 46.
FIGURE 40–7 The fluid mosaic model of membrane structure. The membrane consists of a bimolecular lipid layer with proteins inserted in it or bound to either surface. Integral membrane proteins are firmly embedded in the lipid layers. Some of these proteins completely span the bilayer and are called transmembrane proteins, while others are embedded in either the outer or inner leaflet of the lipid bilayer. Loosely bound to the outer or inner surface of the membrane are the peripheral proteins. Many of the proteins and all the glycolipids have externally exposed oligosaccharide chains. (Reproduced, with permission, from Junqueira LC, Carneiro J: Basic Histology: Text & Atlas, 10th ed., McGraw-Hill, 2003.)
Peripheral proteins do not interact directly with the hydrophobic cores of the phospholipids in the bilayer and thus do not require use of detergents for their release. They are bound to the hydrophilic regions of specific integral proteins and head groups of phospholipids and can be released from them by treatment with salt solutions of high ionic strength. For example, ankyrin, a peripheral protein, is bound to the inner aspect of the integral protein “band 3” of erythrocyte membrane. Spectrin, a cytoskeletal structure within the erythrocyte, is in turn bound to ankyrin and thereby plays an important role in maintenance of the biconcave shape of the erythrocyte.
ARTIFICIAL MEMBRANES MODEL MEMBRANE FUNCTION
Artificial membrane systems can be prepared by appropriate techniques. These systems generally consist of mixtures of one or more phospholipids of natural or synthetic origin that can be treated (eg, by using mild sonication) to form spherical vesicles in which the lipids form a bilayer. Such vesicles, surrounded by a lipid bilayer with an aqueous interior, are termed liposomes (see Figure 15–24).
Some of the advantages and uses of artificial membrane systems in the study of membrane function are as follows:
1. The lipid content of the membranes can be varied, allowing systematic examination of the effects of varying lipid composition on certain functions.
2. Purified membrane proteins or enzymes
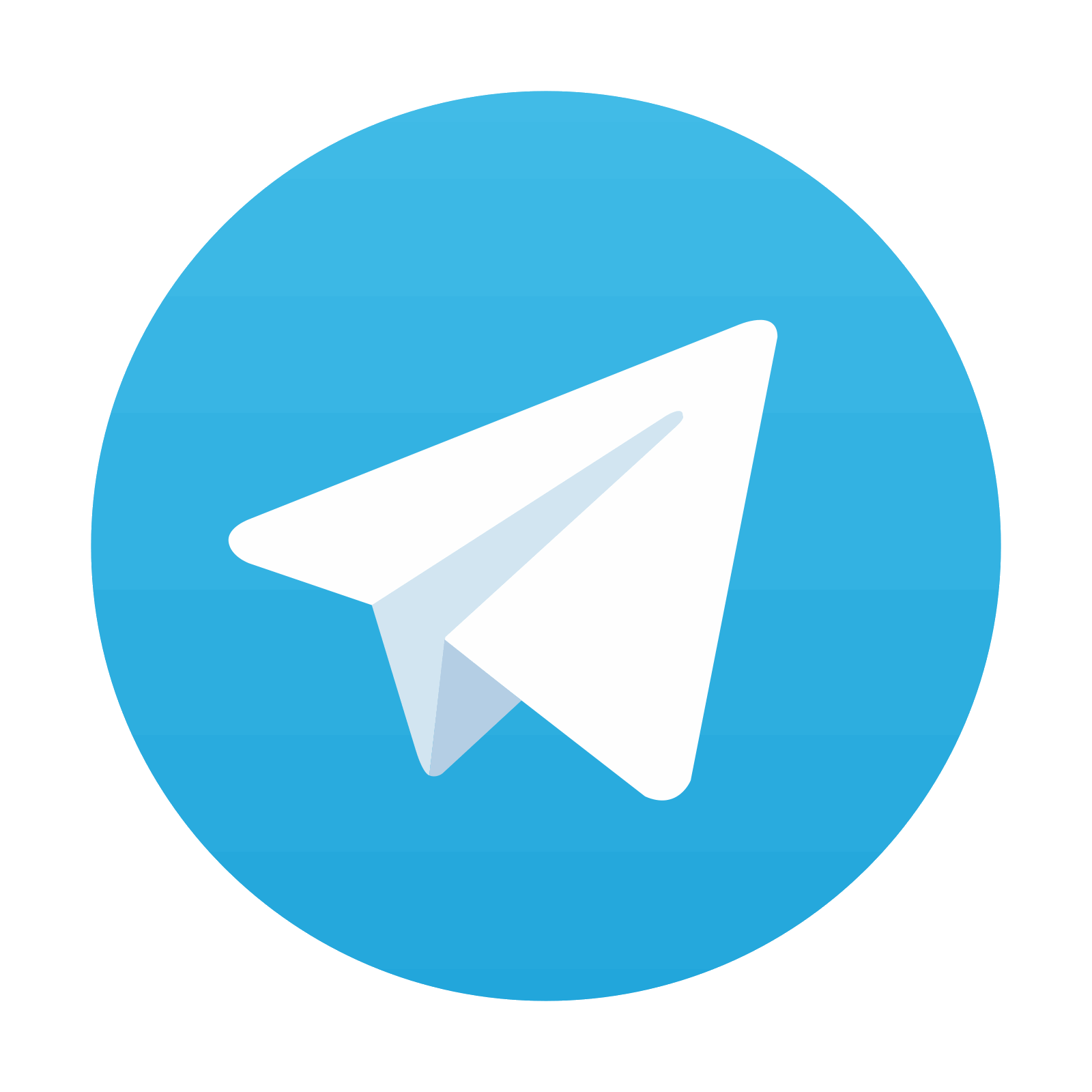
Stay updated, free articles. Join our Telegram channel
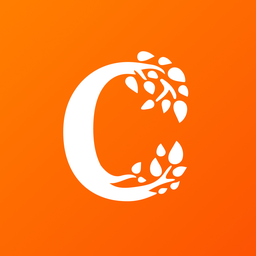
Full access? Get Clinical Tree
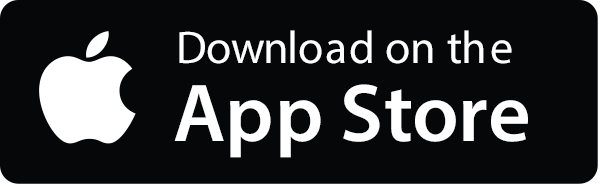
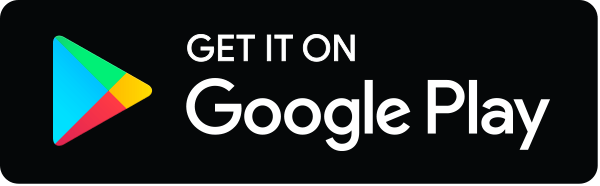