CHAPTER 21 Mechanical Properties of the Lung and Chest Wall: Static and Dynamic
Lung mechanics is the study of the mechanical properties of the lung and the chest wall (which includes the rib cage, diaphragm, abdominal cavity, and anterior abdominal muscles). The primary function of the lung is gas exchange. To achieve this primary function, air must be moved in and out of the lung. The mechanical properties of the lung and chest wall determine the ease or difficulty of this air movement. An understanding of lung mechanics is important to comprehend both how the lung works normally and how the lung works in the presence of disease because almost all lung diseases affect the mechanical properties of the lung. In addition, death from lung disease is almost always due to an inability to overcome the altered mechanical properties of the lung or chest wall, or both. Lung mechanics includes static mechanics (the mechanical properties of a lung whose volume is not changing with time) and dynamic mechanics (properties of a lung whose volume is changing with time). Both are described in this chapter.
STATIC LUNG MECHANICS
Lung Volumes
Clinical evaluation of lung function and the study of static lung mechanics begin with the measurement of lung volumes (Fig. 21-1) and the factors that determine these volumes. All lung volumes are subdivisions of total lung capacity (TLC), the total volume of air that can be contained in the lung. Lung volumes are reported in liters either as volumes or as capacities. A capacity is composed of two or more volumes. Many lung volumes are measured with a spirometer. The patient is asked to first breathe normally into the spirometer, and the volume of air (the tidal volume [VT]) that is moved with each quiet breath is measured. The subject then inhales maximally and exhales forcefully and completely, and the volume of that exhaled air is measured. The total volume of exhaled air, from a maximal inspiration to a maximal exhalation, is the vital capacity (VC). Residual volume (RV) is the air remaining in the lung after a complete exhalation. TLC is the sum of VC and RV; it is the total volume of air contained in the lungs, and it includes the volume of air that can be moved (VC) and the volume of air that is always present (trapped) in the lung (RV). Functional residual capacity (FRC) is the volume of air in the lung at the end of exhalation during quiet breathing and is also called the resting volume of the lung. FRC is composed of RV and the expiratory reserve volume (ERV; the volume of air that can be exhaled from FRC to RV).
Determinants of Lung Volume
Lung volumes are determined by the balance between the lung’s elastic properties and the properties of the muscles of the chest wall. The maximum volume of air contained within the lung and the chest wall (i.e., TLC) is controlled by the muscles of inspiration. With increasing lung volume, the chest wall muscles lengthen progressively. As these muscles lengthen, their ability to generate force decreases. TLC occurs when the inspiratory chest wall muscles are unable to generate the additional force needed to further distend the lung and chest wall. Similarly, the minimal volume of air in the lung (i.e., RV) is controlled by the expiratory muscle force. Decreasing lung volume results in shortening of the expiratory muscles, which in turn results in a decrease in muscle force. The decrease in lung volume is also associated with an increase in the outward recoil pressure of the chest wall. RV occurs when expiratory muscle force is insufficient to further reduce chest wall volume.
Measurement of Lung Volumes
In the helium dilution technique, a known concentration of an inert gas (such as helium) is added to a box of known volume. The box is then connected to a volume that is unknown (the lung volume to be measured). After adequate time for distribution of the inert gas, the new concentration of the inert gas is measured. The change in concentration of the inert gas is then used to determine the new volume in which the inert gas has been distributed (Fig. 21-2). Specifically,
Measurement of lung volumes with a body plethysmograph (body box) uses Boyle’s gas law, which states that pressure multiplied by volume is constant (at a constant temperature). The subject sits in an airtight box (Fig. 21-3) and breathes through a mouthpiece that is connected to a flow sensor (pneumotach). The subject then makes panting respiratory effort against a closed mouthpiece. During the expiratory phase of the maneuver, the gas in the lung becomes compressed, lung volume decreases, and the pressure inside the box falls because the gas volume in the box increases. By knowing the volume of the box and measuring the change in pressure of the box at the mouth, the change in volume of the lung can be calculated. Thus,
where P1 and P2 are mouth pressures and V is FRC.
Lung Compliance
Lung compliance (CL) is a measure of the elastic properties of the lung. It is a measure of how easily the lung is distended. Lung compliance is defined as the change in lung volume resulting from a 1–cm H2O change in the distending pressure of the lung. The units of compliance are mL (or L)/cm H2O. High lung compliance refers to a lung that is readily distended. Low lung compliance, or a “stiff” lung, is a lung that is not easily distended. The compliance of the lung is thus
where ΔV is the change in volume and ΔP is the change in pressure. Graphically, lung compliance is the slope of the line between any two points on the deflation limb of the pressure-volume loop (Fig. 21-4). The compliance of a normal human lung is about 0.2 L/cm H2O, but it varies with lung volume. Note that the lung is less distensible at high lung volumes. For this reason, compliance is corrected for the lung volume at which it is measured (specific compliance) (Fig. 21-5). Changes in lung compliance are associated with certain types of lung disease (e.g., restrictive lung diseases) and are of great clinical importance. Compliance measurements are not often performed for clinical purposes, however, because they require placement of an esophageal balloon. The esophageal balloon, which is connected to a pressure transducer, is an excellent surrogate marker for pleural pressure. The change in pleural pressure (Ppl) is measured as a function of the change in lung volume; that is, CL = ΔV/ΔPpl.
The compliance of the lung is affected by several respiratory disorders. In emphysema, an obstructive lung disease usually of smokers associated with destruction of the alveolar septa and pulmonary capillary bed, the lung is more compliant; that is, for every 1–cm H2O increase in pressure, the increase in volume is greater than in a normal lung (Fig. 21-6). In contrast, in pulmonary fibrosis, a restrictive lung disease associated with increased collagen fiber deposition in the interstitial space, the lung is noncompliant; that is, for every 1–cm H2O change in pressure, the change in volume is less.
Lung–Chest Wall Interactions
The lung and chest wall move together as a unit in healthy people. Separating these structures is the pleural space, which is best thought of as a potential space. Because the lung and chest wall move together, changes in their respective volumes are equal. An understanding of the pressures that surround the lung and chest wall and result in changes in lung volume is essential to comprehend how the lungs work. The pressure changes across the lung and across the chest wall are defined as transmural pressure. For the lung, this transmural pressure is called the transpulmonary (or translung) pressure (PL), and it is defined as the pressure difference between the air spaces (alveolar pressure [PA]) and the pressure surrounding the lung (pleural pressure]Ppl]). That is,
The lung requires positive transpulmonary pressure to increase its volume, and lung volume increases with increasing transpulmonary pressure (Fig. 21-6). The lung assumes its smallest size when transpulmonary pressure is zero. The lung, however, is not totally devoid of air when transpulmonary pressure is zero because of the surface tension–lowering properties of surfactant (see Chapter 20).
The pressure-volume relationships for the lung alone, for the chest wall alone, and for the intact respiratory system are shown in Figure 21-7. A number of important observations can be made by examining the pressure-volume curves of the lung, chest wall, and respiratory system. Note that the transmural pressure across the respiratory system at FRC is zero. At TLC, both lung pressure and chest wall pressure are positive, and they both require positive transmural distending pressure. The resting volume of the chest wall is the volume at which the transmural pressure for the chest wall is zero, and it is approximately 60% of TLC. At volumes greater than 60% of TLC, the chest wall is recoiling inward and positive transmural pressure is needed, whereas at volumes below 60% of TLC, the chest wall tends to recoil outward.
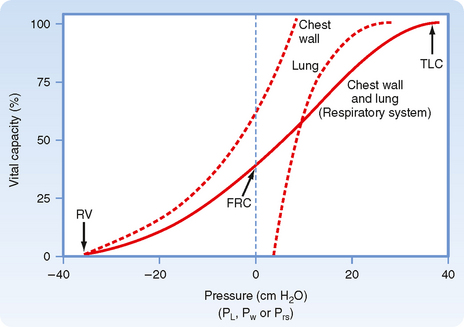
Figure 21-7 Relaxation pressure-volume curve of the lung, chest wall, and respiratory system. The curve for the respiratory system is the sum of the individual curves. The curve for the lung is the same as in Figure 21-6.
The relationship between pleural, alveolar, and elastic recoil pressure is shown in Figure 21-8. Alveolar pressure is the sum of the pleural pressure and elastic recoil pressure of the lung.
Pressure-Volume Relationships
where E is minute ventilation in mL or L/min, VT is tidal volume in mL or L, and f is the frequency or number of breaths per minute.
To understand the relationship between changes in pressure and changes in volume, it is helpful to examine the pressure changes during inspiration and exhalation (Fig. 21-9). In normal individuals during tidal volume breathing, alveolar pressure decreases at the start of inspiration. This decrease in alveolar pressure is usually small (1 to 3 cm H2O). It is much larger in individuals with airway obstruction because of the larger pressure drop that occurs across obstructed airways.
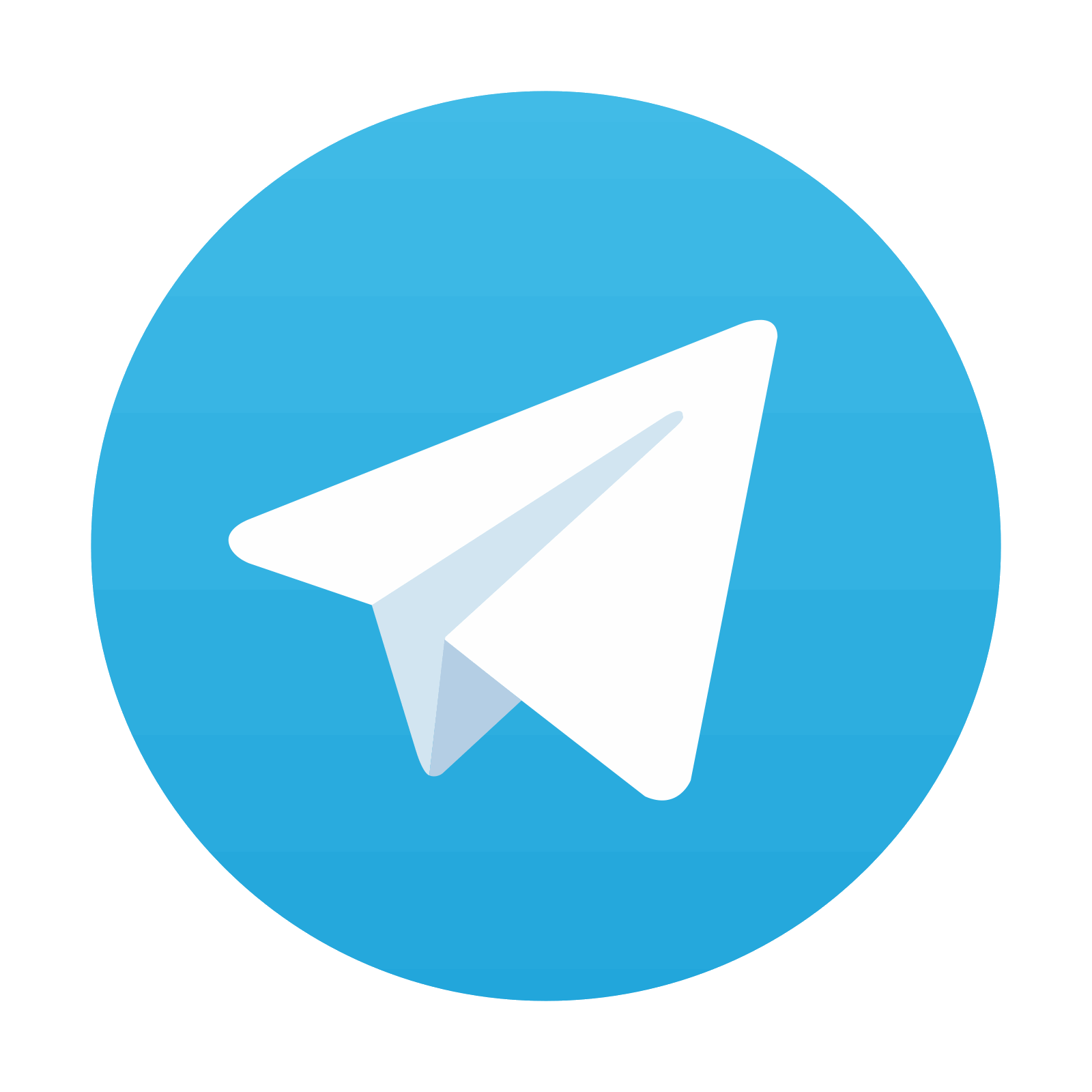
Stay updated, free articles. Join our Telegram channel
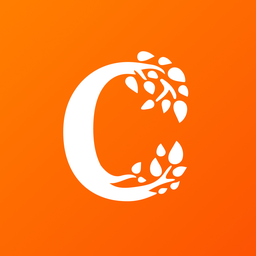
Full access? Get Clinical Tree
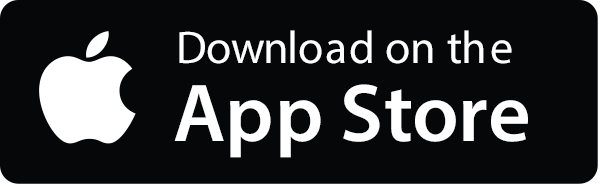
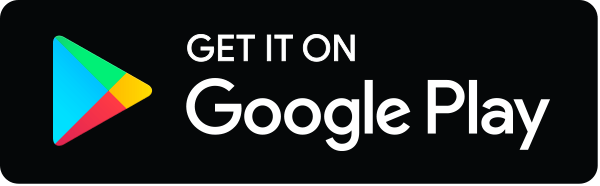