Elizabeth N. Pearce, MD, MSc, and Hedley C. Freake, PhD Inorganic iodine occurs predominantly in nature in the form of the anion iodide. Its sole function in humans and other mammals is the synthesis of thyroid hormones. These iodinated derivatives of the amino acid tyrosine, shown in Figure 38-1, are thyroxine, or 3,5,3′,5′-tetraiodothyronine (T4), and 3,5,3′-triiodothyronine (T3). Because they are derived from amino acids, thyroid hormones are in the L-form. D-Isomers can be synthesized but have lower biological activity. Iodine is a reasonably abundant element, but its solubility leads to wide regional variations in its availability. The iodine content of soils is diminished by exposure to rain, snow, and glaciation, which leach out the mineral and deposit it in the oceans. Although iodine is volatilized from the sea and returns to land through rainwater, this does not make up for the long-term loss of iodine from older exposed soils. Iodine-deficient areas include mountainous regions, such as the Himalayas, Andes, and Alps, and also river deltas, such as the Ganges and the Yellow River, where frequent flooding has leached out the mineral (Zimmermann, 2009). The iodine content of most food sources is low. The iodine content of plants averages 1 mg/kg dry weight, but it may be only 1% of that amount in plants grown in iodine-deficient areas. Its content in animal foods, including milk, reflects the amount found in the feeds supplied to the animals. Foods arising from the sea, such as fish and seaweed, provide a rich source. Foods of marine origin have greater amounts of iodine because marine animals can concentrate iodine from seawater. In more developed parts of the world, the food available to a particular community is usually drawn from a variety of geographical locations and thus, in aggregate, is less likely to be deficient in iodine. However, supplementation, particularly in the form of iodized salt as has been used in the United States and many other parts of the world, is the only reliable way to ensure an adequate dietary supply in low-iodine areas. This relatively simple public health program has reaped enormous benefits. Iodine intakes may also be increased by the use of iodine-containing products at various points in the food production process. For example, iodates are used in bread making, and iodine-containing antiseptics are used in dairy facilities (Pearce et al., 2004). Thus processed foods may contain enhanced levels of iodine from the addition of iodized salt or other additives. Iodine may be found in different forms in foods, including iodide (I–) and iodate (IO3–), the compound added to bread. Dietary iodine is reduced to iodide and absorbed efficiently along the length of the gastrointestinal tract, especially in the upper portion. The adult human body normally contains 15 to 20 mg of iodine, and about three fourths of this is found in the thyroid gland. The thyroid actively takes up iodide via the sodium/iodide symporter (NIS), which can be inhibited by environmental contaminants. Concentration of iodine in the gland is 40 times that in plasma under normal circumstances and can become still higher in iodine deficiency. Iodine accumulates to a much lesser extent in other tissues such as the salivary glands, stomach, ovaries, and testes. The kidneys are the other principal site for iodine removal from the circulation. They are incapable of conserving the mineral, and hence the kidneys represent the main route for excretion. The amount of iodine found in urine is proportional to the plasma concentration and can be used as a convenient index of iodine status in populations. Iodide is actively secreted into breast milk via NIS in lactating women. Small amounts of iodine are also lost in feces and sweat. Figure 38-2 provides a summary of the routes of iodine in the body from ingestion to excretion. The process of thyroid hormone synthesis is illustrated in Figure 38-3. Iodide is actively transported across the basolateral membrane into the thyroid follicular cell cytoplasm by the NIS. It traverses the thyroid cells and is passively translocated into the follicular lumen by pendrin. Cloned in 1997, pendrin is a highly hydrophobic glycoprotein containing 780 amino acids. It is composed of 12 transmembrane domains and a carboxy-terminus inside the cytosol (Royaux et al., 2000). Pendrin is expressed not only in thyrocytes but also in kidney and inner ear cells. The apical iodide transporter (AIT), the cystic fibrosis transmembrane conductance regulator (CFTR), SLC5A8 (a member of the solute carrier 5A family), and the chloride channel 5 (ClCn5) are other putative transport proteins involved in iodide efflux across the apical membrane into the follicular lumen that have yet to be fully characterized. likely to be eaten in sufficient quantities to cause concern. Goitrogens work either by competitively inhibiting iodide uptake by the thyroid gland or by blocking its incorporation into the tyrosyl residues of thyroglobulin and their subsequent condensation. Antithyroid drugs, such as propylthiouracil and methimazole, work by the latter mechanism and are used clinically in the treatment of hyperthyroidism. Thyroid hormones are carried in the blood by three proteins: thyroid-binding globulin, thyroid-binding prealbumin, and albumin. Prealbumin is also known as transthyretin and functions with retinol-binding protein in retinol transport (see Chapter 30). More than 99% of both T4 and T3 circulate bound to these proteins, but T4 is bound 10 times more tightly than T3. It appears likely that only the small free fraction is available for tissue uptake, either to exert biological activity or for further metabolism. This tight binding means that thyroid hormones, particularly T4, have relatively long plasma half-lives (6 to 8 days) and also that there is a large plasma pool of thyroid hormones, which can become available to tissues after dissociation from the binding proteins. The T4 plasma concentration in normal humans is approximately 100 nmol/L, which is 50- to 100-fold higher than that for T3. The relative abundance of T4 reflects both that T4 is the primary product of the thyroid gland and that it has a longer plasma half-life than does T3 (1 day). Recent research has shown that thyroid hormone transport across cell membranes is energy dependent and mediated by specific organic anion and amino acid transporters, including the sodium taurocholate cotransporting polypeptide (NTCP), various sodium-independent organic anion transporting polypeptides, L-type amino acid transporters (LAT1 and LAT2), the fatty acid translocase (FAT, CD36), and the monocarboxylate transporters (MCT) 8 and 10. Congenital defects in MCT result in severe neurological and cognitive impairments (Heuer and Visser, 2009). T4, the predominant circulating thyroid hormone, is really a prohormone that requires deiodination at the 5′ position in the outer ring to generate the biologically active T3. Deiodination serves not only to activate thyroid hormones but also to deactivate them (Figure 38-4). Removal of iodine from the inner ring of T4 will result in the production of reverse T3 (rT3; 3,3′,5′-triiodothyronine), and similar processing of T3 produces 3,3′-diiodothyronine, both of which are inactive metabolites. A family of microsomal enzymes is responsible for deiodination (Bianco et al., 2002). The type 1 deiodinase is found in liver, kidney, and thyroid gland. It is associated with the plasma membrane and is thought to contribute significantly to circulating T3 concentrations. It can perform inner ring as well as outer ring deiodinations, and its preferred substrate is rT3. Type 1 deiodinase activity is increased in hyperthyroidism and decreased in the hypothyroid state. The type 2 deiodinase, located in brain, pituitary, and brown adipose tissue, operates solely on the outer ring. It is located in the endoplasmic reticulum and specifically functions to convert T4 to T3 for local use within these tissues. However, it is now appreciated that type 2 deiodinase can also contribute significantly to circulating levels of T3 in plasma. In tissues containing the type 2 enzyme, thyroid hormone status will depend primarily on plasma levels of T4 rather than T3. The activity of this enzyme is increased in the hypothyroid state, which means that these tissues may be partially protected from the effects of hypothyroidism by this local production. Genes encoding all three enzymes have now been cloned in multiple species and this has allowed many insights into the functioning and regulation of this enzyme family (Bianco et al., 2002). Of particular interest is the discovery that all three enzymes contain the unusual amino acid selenocysteine at their active site. This amino acid is similar to cysteine, except that the sulfur is substituted by selenium (see Chapter 39). The nucleotide triplet UGA encodes this substitution, which is critical for the catalytic properties of the enzyme. Under most circumstances this triplet is read as a stop codon but other sequences within the deiodinase messenger RNAs (mRNAs) override this coding and dictate the incorporation of selenocysteine. This linkage between thyroid hormone deiodination and selenium explains earlier work in rats, which showed that selenium deficiency reduced plasma T3 and thereby impaired thyroid hormone status. From the preceding discussion it is apparent that thyroid hormone status can be modified at a number of different levels. The primary site may be output from the thyroid gland, but the rate of conversion of the prohormone to active T3 is also important. Under normal circumstances, circulating thyroid hormone levels are tightly regulated because of a negative feedback loop controlling their production (Figure 38-5). Thyrotroph cells in the anterior pituitary produce thyrotropin, or thyroid-stimulating hormone (TSH), a glycoprotein that is a heterodimer of two subunits encoded by separate genes. The α-subunit of TSH is similar to that of the gonadotropic pituitary hormones (luteinizing hormone and follicle-stimulating hormone), whereas the β-subunit is unique. TSH acts on the thyroid gland, primarily by a cyclic AMP–mediated mechanism, to stimulate iodide uptake and organification and release of thyroid hormones. T4 travels back to the pituitary, where it is deiodinated by the type 2 enzyme. The T3 that is produced operates at a transcriptional level to inhibit the production of TSH, thereby completing the cycle. TSH is also under the control of the hypothalamic tripeptide thyrotropin-releasing hormone (TRH), which is responsible for basal production of TSH by the pituitary. It provides a mechanism by which thyroid hormone status can be modulated at a central level, either positively (e.g., in response to cold) or negatively (e.g., in response to stress or illness). T3 has also been shown to inhibit transcription of the TRH gene, meaning that the negative feedback loop extends up to the hypothalamus. Most and perhaps all actions of thyroid hormones are mediated by effects within the nucleus. This mechanism is outlined in Figure 38-6. Following dissociation from plasma proteins, T4 and T3 enter the cell. The T4 is deiodinated and T3 enters the nucleus. There it is bound by specific nuclear receptor proteins, which are associated with a set of target genes (Box 38-1). Hormone binding to these receptors alters these associations in a manner that leads to a change in the rate of transcription of the target genes. Changes in gene transcription in turn lead to changed amounts of mRNA, which are translated to correspondingly different amounts of protein. Thereby the physiological state is altered. Enormous progress has been made in the last 20 years in understanding the mechanism of action of thyroid hormone on a molecular level (Cheng et al., 2010). An important starting point for these advances was the cloning in 1986 of complementary DNAs (cDNAs) encoding thyroid hormone receptors (TRs) in two independent laboratories (Sap et al., 1986; Weinberger et al., 1986). The realization that TRs are part of a family of nuclear acting proteins has facilitated progress in understanding their mechanism of action, because analogies can be drawn with other members of the class (Aranda and Pascual, 2001). This family includes receptors not only for steroid hormones but also for retinoic acid, 1,25-dihydroxyvitamin D, fatty acids, steroids, and a host of other lesser known or uncharacterized ligands. These receptors have a modular structure (Figure 38-7). They all have a carboxy-terminal region that binds to the ligand, and this region provides each receptor with its hormone ligand specificity. The area that is most highly conserved across the family is the DNA-binding region, through which the receptors recognize and bind to their target genes. This region contains conserved cysteine residues that coordinate two atoms of zinc. These stabilize a conformation required for binding to target genes. Regions that appear to be required for nuclear localization of the protein, that facilitate dimerization between receptors, or that are required for communicating the signal for altered transcription have also been described.
Iodine
Production and Metabolism of Thyroid Hormones
Use of Iodine: Thyroid Hormones
Dietary Sources of Iodine
Absorption, Storage, and Excretion of Iodine
Synthesis of Thyroid Hormones
Circulation
Transport
Activation
Regulation of Thyroid Hormone Status
Mechanism of Action of Thyroid Hormones
Nuclear Receptors
Functional Regions of Receptors
Stay updated, free articles. Join our Telegram channel
Full access? Get Clinical Tree
Iodine
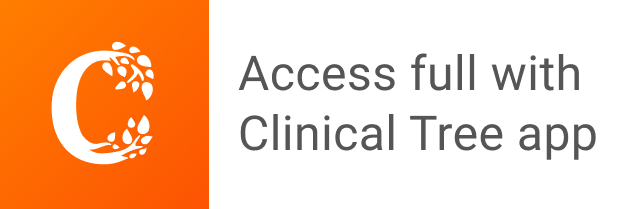