CHAPTER 40 Introduction to the Cell Cycle
The cell cycle, is the series of events that leads to the duplication and division of a cell. Research on the molecular events of cell-cycle control revealed that variations of similar mechanisms operate the cell cycles of all eukaryotes from yeasts to humans. Furthermore, the components that regulate cell growth and division also play key roles in the cessation of cell division that accompanies cell differentiation. Control of the cell cycle is of major importance to human health because cancer, which is usually caused by perturbations of cell-cycle regulation, affects 46% of males and 38% of females in the United States.
Although animal cells have a wide variety of specialized cell cycles, the cells in the stratified epithelium that forms skin illustrate the most common lifestyles (Fig. 40-1). The basal layer of the epithelium is composed of stem cells that divide only occasionally. (See Box 41-1.) They can activate the cell cycle on demand and then return to a nondividing state. When specific signals induce stem cells to proliferate, one daughter cell usually remains a stem cell and the other enters a pool of rapidly dividing cells. These dividing cells populate the upper layers of the epithelium, stop dividing, and gradually differentiate into the specialized cells that cover the surface.
Similarly, the nervous system contains a few stem cells and a few dividing cells, but most neurons, once differentiated, can live for more than 100 years without dividing again. Like stem cells, fibroblasts of the connective tissue (see Fig. 28-2) are typically nondividing, but they can be stimulated to enter the cell cycle following wounding or other stimuli (see Fig. 32-11).
Principles of Cell-Cycle Regulation
The goal of the cell cycle in most cases is to produce two daughter cells that are accurate copies of the parent (Fig. 40-2). The cell cycle integrates a continuous growth cycle (the increase in cell mass) with a discontinuous division or chromosome cycle (the replication and partitioning of the genome into two daughter cells). The chromosome cycle is driven by a sequence of enzymatic cascades that produce a sequence of discrete biochemical “states” of the cytoplasm. Each state arises by destruction or inactivation of key enzymatic activities characteristic of the preceding state and expression or activation of a new cohort of activities. Later sections of this chapter explain these mechanisms.
Phases of the Cell Cycle
In thinking about the cell cycle, it is convenient to divide the process into a series of phases. Recognition of cell-cycle phases began in 1882, when Flemming named the process of nuclear division mitosis (from the Greek mito, or “thread”) after the appearance of the condensed chromosomes. It initially appeared that cells were active only during mitosis, so the rest of the cell cycle was called interphase (or resting stage) (Box 40-1).
Once DNA was recognized as the agent of heredity in the 1940s, it was deduced that DNA must be duplicated at some time during interphase so that daughter cells can each receive a full complement of genetic material. A key experiment identified the relationship between the timing of DNA synthesis and the mitotic cycle (Fig. 40-3) and defined the four cell-cycle phases as they are known today (Fig. 40-2).
S Phase
Chromosomes of higher eukaryotes are so large that replication of the DNA must be initiated at many different sites, termed origins of replication. In budding yeast, the approximately 400 origins are spaced an average of 30,000 base pairs apart. An average human chromosome contains about 150 ×106 base pairs of DNA, about 10 times the size of the entire budding yeast genome, so many more origins are required. Each region of the chromosome that is replicated from a single origin is referred to as a replicon.
During replication, the duplicated DNA molecules, called sister chromatids, become linked to each other by a protein complex called cohesin (see Fig. 13-19). This pairing of sister chromatids is important for their symmetrical segregation later in mitosis (see Fig. 44-16).
M Phase
Prometaphase begins when the nuclear envelope breaks down (in higher eukaryotes) and chromosomes begin to attach randomly to microtubules emanating from the two poles of the forming mitotic spindle. Chromosomes may also nucleate some spindle microtubules. Once both kinetochores on a pair of sister chromatids are attached to opposite spindle poles, the chromosome slowly moves to a point midway between the poles. When all chromosomes are properly attached, the cell is said to be in metaphase.
Checkpoints
The cell cycle is highly regulated, and checkpoints control transitions between cell-cycle stages. Checkpoints are biochemical circuits that detect external or internal problems and send inhibitory signals to the cell-cycle system. There are four major types of checkpoints. The restriction point in the G1 phase is sensitive to the physiological state of the cell and to its interactions with the surrounding extracellular matrix. Cells that do not receive appropriate growth stimuli from their environment do not progress past this point in the G1 phase and may commit suicide by apoptosis (see Chapter 46).
The checkpoints in G1, S, and G2 use common strategies to regulate cell-cycle progression (Fig. 40-4). DNA damage is detected by sensors. These activate transducers, which are often protein kinases but may also be transcriptional activators. The transducers act on effectors, which ultimately block cell-cycle progression and may also fulfill other functions. Two key protein kinases, ataxia-telangiectasia mutated (ATM) and ataxia-telangiectasia and Rad9 related (ATR), lie at the head of the pathway and may act as sensors of DNA damage. They activate two transducer kinases Chk1 and Chk2 and also stabilize a transcription factor called p53 that induces the expression of a cohort of genes involved in halting cell-cycle progression as well as genes that trigger cell death by apoptosis. Chapters 41 and 43 discuss these proteins in detail. In general, DNA damage checkpoints block cell-cycle progression by inhibiting the cyclin-dependent kinases by a variety of mechanisms.
The Biochemical Basis of Cell-Cycle Transitions
Cyclin-Dependent Kinases
Genetic analysis of the cell cycle in the fission yeast Schizosaccharomyces pombe identified a gene called cell division cycle–2+ (cdc2+) that is essential for cell-cycle progression during both the G1 S and G2
M transitions (Box 40-2). The product of this gene, a protein kinase of 34,000D originally called p34cdc2, is the prototype for a family of protein kinases that is crucial for cell-cycle progression in all eukaryotes. This mechanism of cell-cycle control is so well conserved that a human homolog of p34cdc2 can replace the yeast protein, restoring a normal cell cycle to a cdc2 mutant yeast. Boxes 40-3 and 40-4 present a number of the key experiments and experimental systems that led to the identification of the molecules that drive the cell cycle.
BOX 40-2 Use of Genetics to Study the Cell Cycle
Studies of the distantly related budding and fission yeasts Saccharomyces cerevisiae and Schizosaccharomyces pombe (see Fig. 2-9) have been extremely important for understanding the cell cycle for several reasons. First, the proteins that control the cell cycle are remarkably conserved between yeasts and mammals. Second, both yeast genomes are sequenced and annotated, simplifying characterization of novel gene products. Third, genetic analysis is facilitated, as both yeasts grow as haploids, and both efficiently incorporate cloned DNA into their chromosomes by homologous recombination.
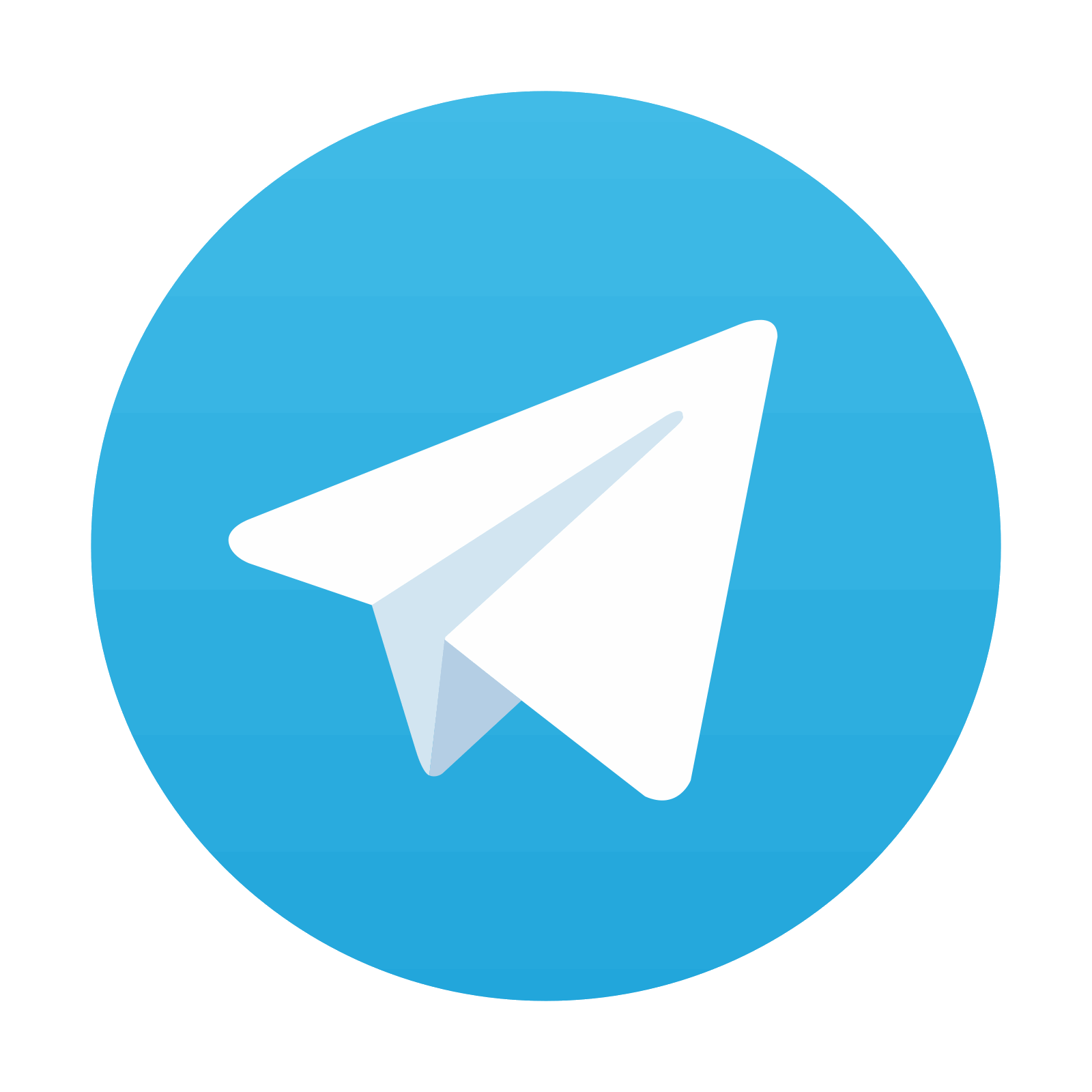
Stay updated, free articles. Join our Telegram channel
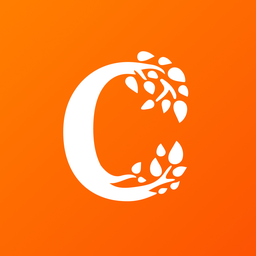
Full access? Get Clinical Tree
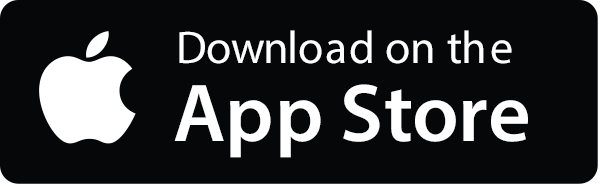
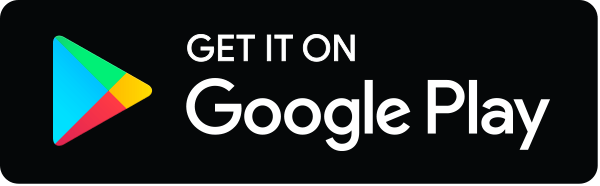