This chapter summarizes and integrates the major pathways for the utilization of carbohydrates and fats as fuels. We concentrate on reviewing the regulatory mechanisms that determine the flux of metabolites in the fed and fasting states, integrating the pathways that were described separately under carbohydrate and lipid metabolism. The next section of the book covers the mechanisms by which the pathways of nitrogen metabolism are coordinated with fat and carbohydrate metabolism.
For the species to survive, it is necessary for us to store excess food when we eat and to use these stores when we are fasting. Regulatory mechanisms direct compounds through the pathways of metabolism involved in the storage and utilization of fuels. These mechanisms are controlled by hormones, by the concentration of available fuels, and by the energy needs of the body.
Changes in hormone levels, in the concentration of fuels, and in energy requirements affect the activity of key enzymes in the major pathways of metabolism. Intracellular enzymes are generally regulated by activation and inhibition, by phosphorylation and dephosphorylation (or other covalent modifications), by induction and repression of synthesis, and by degradation. Activation and inhibition of enzymes cause immediate changes in metabolism. Phosphorylation and dephosphorylation of enzymes affect metabolism slightly less rapidly. Induction and repression of enzyme synthesis are much slower processes, usually affecting metabolic flux over a period of hours. Degradation of enzymes decreases the amount available to catalyze reactions.
The pathways of metabolism have multiple control points and multiple regulators at each control point. The function of these complex mechanisms is to produce a graded response to a stimulus and to provide sensitivity to multiple stimuli so that an exact balance is maintained between flux through a given step (or series of steps) and the need or use for the product. Pyruvate dehydrogenase (PDH) is an example of an enzyme that has multiple regulatory mechanisms. Regardless of insulin levels, the enzyme cannot become fully activated in the presence of products and the absence of substrates.
The major hormones that regulate the pathways of fuel metabolism are insulin and glucagon. In liver, all effects of glucagon are reversed by insulin, and some of the pathways that insulin activates are inhibited by glucagon. Thus, the pathways of carbohydrate and lipid metabolism are generally regulated by changes in the insulin/glucagon ratio.
Although glycogen is a critical storage form of fuel because blood glucose levels must be carefully maintained, adipose triacylglycerols are quantitatively the major fuel store in the human. After a meal, both dietary glucose and fat are stored in adipose tissue as triacylglycerol. This fuel is released during fasting, when it provides the main source of energy for the tissues of the body. The length of time we can survive without food depends mainly on the size of our bodies’ fat stores.
THE WAITING ROOM 
Within 2 months of her surgery to remove a benign insulin-secreting β-cell tumor of the pancreas, Connie C. (see Chapter 19) was again jogging lightly. She had lost the 8 lb that she had gained in the 6 weeks before her surgery. Because her hypoglycemic episodes were no longer occurring, she had no need to eat frequent carbohydrate snacks to prevent the adrenergic and neuroglycopenic symptoms that she had experienced when her tumor was secreting excessive amounts of insulin.
A few months after her last hospitalization, Dianne A. was once again brought to the hospital emergency room in diabetic ketoacidosis (DKA). Blood samples for glucose and electrolytes were drawn repeatedly during the first 24 hours. The hospital laboratory reported that the serum in each of these specimens appeared opalescent rather than having its normal clear or transparent appearance. This opalescence results from light scattering caused by the presence of elevated levels of triacylglycerol-rich lipoproteins in the blood.
When Deborah S. initially presented (see Chapter 19) with type 2 diabetes mellitus at age 39, she was approximately 30 lb over her ideal weight. Her high serum glucose levels were accompanied by abnormalities in her 14-hour fasting lipid profile. Her serum total cholesterol, low-density lipoprotein (LDL) cholesterol, and triacylglycerol levels were elevated, and her serum high-density lipoprotein (HDL) cholesterol level was below the normal range.
I. Regulation of Carbohydrate and Lipid Metabolism in the Fed State
A. Mechanisms That Affect Glycogen and Triacylglycerol Synthesis in Liver
After a meal, the liver synthesizes glycogen and triacylglycerol. The level of glycogen stored in the liver can increase from approximately 80 g after an overnight fast to a limit of approximately 200 to 300 g. Although the liver synthesizes triacylglycerol, it does not store this fuel, but rather packages it in very-low-density lipoprotein (VLDL) and secretes it into the blood. The fatty acids of the VLDL triacylglycerols secreted from the liver are stored as adipose triacylglycerols. Adipose tissue has an almost infinite capacity to store fat, limited mainly by the ability of the heart to pump blood through the capillaries of the expanding adipose mass. Although we store fat throughout our bodies, it tends to accumulate in places where it does not interfere too much with our mobility: in the abdomen, hips, thighs, and buttocks.
Both the synthesis of liver glycogen and the conversion by the liver of dietary glucose to triacylglycerol (lipogenesis) are regulated by mechanisms involving key enzymes in these pathways.
1. Glucokinase
After a meal, glucose can be converted to glycogen or to triacylglycerol in the liver. For both processes, glucose is first converted to glucose 6-phosphate by glucokinase, a liver enzyme that has a high Km for glucose (Fig. 34.1). Because of the enzyme’s low affinity for glucose, this enzyme is most active in the fed state, when the concentration of glucose is particularly high because the hepatic portal vein carries digestive products directly from the intestine to the liver. Synthesis of glucokinase is also induced by insulin (which is elevated after a meal) and repressed by glucagon (which is elevated during fasting). In keeping with the liver’s function in maintaining blood glucose levels, this system is set up such that the liver can metabolize glucose only when sugar levels are high and not when sugar levels are low.
FIGURE 34.1 Regulation of glucokinase, phosphofructokinase-1 (PFK-1), and pyruvate kinase in the liver. ADP, adenosine diphosphate; AMP, adenosine monophosphate; ATP, adenosine triphosphate; cAMP, cyclic adenosine monophosphate; F-1,6-BP, fructose 1,6-bisphosphate; F-2,6-BP, fructose 2,6-bisphosphate.
2. Glycogen Synthase
In the conversion of glucose 6-phosphate to glycogen, the key regulatory enzyme is glycogen synthase. This enzyme is activated by dephosphorylation, which occurs when insulin is elevated and glucagon is decreased (Fig. 34.2) and by the increased level of glucose.
FIGURE 34.2 Regulation of glycogen synthase. This enzyme is phosphorylated by a series of kinases, which are initiated by the cyclic adenosine monophosphate (cAMP)-dependent protein kinase under fasting conditions. It is dephosphorylated and active after a meal, and glycogen is stored. , phosphate; ⊕, activated by; ADP, adenosine diphosphate; ATP, adenosine triphosphate; Glucose-1-P, glucose 1-phosphate; glucose-6-P, glucose 6-phosphate; Pi, inorganic phosphate; PPi, pyrophosphate; UDP, uridine diphosphate; UTP, uridine triphosphate.
3. Phosphofructokinase-1 and Pyruvate Kinase
For lipogenesis, glucose 6-phosphate is converted through glycolysis to pyruvate. Key enzymes that regulate this pathway in the liver are phosphofructokinase-1 (PFK-1) and pyruvate kinase. PFK-1 is allosterically activated in the fed state by fructose 2,6-bisphosphate and adenosine monophosphate (AMP) (see Fig. 34.1). Phosphofructokinase-2 (PFK-2), the enzyme that produces the activator fructose 2,6-bisphosphate, is dephosphorylated and the kinase activity is active after a meal (see Chapter 22). Pyruvate kinase is also activated by dephosphorylation, which is stimulated by the increase of the insulin/glucagon ratio in the fed state (see Fig. 34.1).
4. Pyruvate Dehydrogenase and Pyruvate Carboxylase
The conversion of pyruvate to fatty acids requires a source of acetyl coenzyme A (acetyl CoA) in the cytosol. Pyruvate can only be converted to acetyl CoA in mitochondria, so it enters mitochondria and forms acetyl CoA through the PDH reaction. This enzyme is dephosphorylated and most active when its supply of substrates and adenosine diphosphate (ADP) is high, its products are used, and insulin is present (see Fig. 23.13).
Pyruvate is also converted to oxaloacetate. The enzyme that catalyzes this reaction, pyruvate carboxylase, is activated by acetyl CoA. Because acetyl CoA cannot cross the mitochondrial membrane directly to form fatty acids in the cytosol, it condenses with oxaloacetate, producing citrate. The citrate that is not required for tricarboxylic acid (TCA) cycle activity crosses the membrane and enters the cytosol.
As fatty acids are produced under conditions of high energy, the high NADH/NAD+ ratio in the mitochondria inhibits isocitrate dehydrogenase, which leads to citrate accumulation within the mitochondrial matrix. As the citrate accumulates, it is transported out into the cytosol to donate carbons for fatty acid synthesis.
5. Citrate Lyase, Malic Enzyme, and Glucose 6-Phosphate Dehydrogenase
In the cytosol, citrate is cleaved by citrate lyase, an inducible enzyme, to form oxaloacetate and acetyl CoA (Fig. 34.3). The acetyl CoA is used for fatty acid biosynthesis and for cholesterol synthesis, pathways that are activated by insulin. Oxaloacetate is recycled to pyruvate via cytosolic malate dehydrogenase and malic enzyme, which is inducible. Malic enzyme generates NADPH for the reactions of the fatty acid synthase complex. NADPH is also produced by the two enzymes of the pentose phosphate pathway (see Chapter 27): glucose 6-phosphate dehydrogenase and 6-phosphogluconate dehydrogenase. Glucose 6-phosphate dehydrogenase is also induced by insulin.
FIGURE 34.3 Regulation of citrate lyase, malic enzyme, glucose 6-phosphate dehydrogenase, and fatty acid synthase. Citrate lyase, which provides acetyl coenzyme A (acetyl CoA) for fatty acid biosynthesis, the enzymes that provide NADPH (malic enzyme, glucose 6-phosphate dehydrogenase), as well as fatty acid synthase, are inducible (↑). ADP, adenosine diphosphate; ATP, adenosine triphosphate; FA, fatty acid; malonyl CoA, malonyl coenzyme A; NAD, nicotinamide adenine dinucleotide; OAA, oxaloacetate; Pi, inorganic phosphate.
6. Acetyl CoA Carboxylase
Acetyl CoA is converted to malonyl CoA, which provides the two-carbon units for elongation of the growing fatty acyl chain on the fatty acid synthase complex. Acetyl CoA carboxylase, the enzyme that catalyzes the conversion of acetyl CoA to malonyl CoA, is controlled by three of the major mechanisms that regulate enzyme activity (Fig. 34.4). It is activated by citrate, which causes the enzyme to polymerize, and inhibited by long-chain fatty acyl CoA. A phosphatase stimulated by insulin activates the enzyme by dephosphorylation. The third means by which this enzyme is regulated is induction: the quantity of the enzyme increases in the fed state.
FIGURE 34.4 Regulation of acetyl CoA carboxylase (ACC). ACC is regulated by activation and inhibition, by phosphorylation (mediated by the adenosine monophosphate [AMP]-activated protein kinase) and dephosphorylation (via an insulin-stimulated phosphatase), and by induction (↑) and repression. It is active in the fed state. ADP, adenosine diphosphate; ATP, adenosine triphosphate; malonyl CoA, malonyl coenzyme A; Palmitoyl CoA, palmitoyl coenzyme A; Pi, inorganic phosphate.
Malonyl CoA, the product of the acetyl CoA carboxylase reaction, provides the carbons for the synthesis of palmitate on the fatty acid synthase complex. Malonyl CoA also inhibits carnitine:palmitoyltransferase I (CPTI, also known as carnitine:acyltransferase I), the enzyme that prepares long-chain fatty acyl CoA for transport into mitochondria (Fig. 34.5). In the fed state, when acetyl CoA carboxylase is active and malonyl CoA levels are elevated, newly synthesized fatty acids are converted to triacylglycerols for storage rather than being transported into mitochondria for oxidation and formation of ketone bodies.
FIGURE 34.5 Inhibition of transport of fatty acids (FA) into mitochondria by malonyl coenzyme A (malonyl CoA). In the fed state, malonyl CoA (the substrate for fatty acid synthesis produced by acetyl coenzyme A [acetyl CoA] carboxylase) is elevated. It inhibits carnitine palmitoyltransferase I (CPTI), preventing the transport of long-chain fatty acids into mitochondria. Therefore, substrate is not available for β-oxidation and ketone body synthesis. CoA, coenzyme A; CPTII, carnitine palmitoyltransferase II.
7. Fatty Acid Synthase Complex
In a well-fed individual, the quantity of the fatty acid synthase complex is increased (see Fig. 34.3). The genes that produce this enzyme complex are induced by increases in the insulin/glucagon ratio. The amount of the complex increases slowly after a few days of a high-carbohydrate diet.
Glucose 6-phosphate dehydrogenase, which generates NADPH in the pentose phosphate pathway, and malic enzyme, which produces NADPH, are also induced by the increase of insulin.
The palmitate produced by the synthase complex is converted to palmitoyl CoA and elongated and desaturated to form other fatty acyl CoA molecules, which are converted to triacylglycerols. These triacylglycerols are packaged and secreted into the blood as VLDL.
B. Mechanisms That Affect the Fate of Chylomicrons and VLDL
The lipoprotein triacylglycerols in chylomicrons and VLDL are hydrolyzed to fatty acids and glycerol by lipoprotein lipase (LPL), an enzyme attached to endothelial cells of capillaries in muscles and adipose tissues. The enzyme found in muscle, particularly heart muscle, has a low Km for these blood lipoproteins. Therefore, it acts even when these lipoproteins are present at very low concentrations in the blood. The fatty acids enter muscle cells and are oxidized for energy. The enzyme found in adipose tissue has a higher Km and is most active after a meal when blood lipoprotein levels are elevated.
C. Mechanisms That Affect Triacylglycerol Storage in Adipose Tissue
Insulin stimulates adipose cells to synthesize and secrete LPL, which hydrolyzes the chylomicron and VLDL triacylglycerols. Apolipoprotein CII, donated to chylomicrons and VLDL by HDL, activates LPL (Fig. 34.6).
FIGURE 34.6 Regulation of the storage of triacylglycerols (TG) in adipose tissue. Insulin stimulates the secretion of lipoprotein lipase (LPL) from adipose cells and the transport of glucose into these cells. ApoCII activates LPL. CII, apolipoprotein CII; CoA, coenzyme A; DHAP, dihydroxyacetone phosphate; FA, fatty acids; HDL, high-density lipoprotein; IDL, intermediate-density lipoprotein; LDL, low-density lipoprotein; VLDL, very-low-density lipoprotein.
Fatty acids released from chylomicrons and VLDL by LPL are stored as triacylglycerols in adipose cells. The glycerol released by LPL is not used by adipose cells because they lack glycerol kinase. Glycerol can be used by liver cells, however, because these cells do contain glycerol kinase. In the fed state, liver cells convert glycerol to the glycerol moiety of the triacylglycerols of VLDL, which is secreted from the liver to distribute the newly synthesized triglycerides to the tissues.
Insulin causes the number of glucose transporters in adipose cell membranes to increase. Glucose enters these cells and is oxidized, producing energy and providing the glycerol 3-phosphate moiety for triacylglycerol synthesis (via the dihydroxyacetone phosphate intermediate of glycolysis).
II. Regulation of Carbohydrate and Lipid Metabolism during Fasting
A. Mechanisms in Liver That Serve to Maintain Blood Glucose Levels
During fasting, the insulin/glucagon ratio decreases. Liver glycogen is degraded to produce blood glucose because enzymes of glycogen degradation are activated by cAMP-directed phosphorylation (see Fig. 26.7). Glucagon stimulates adenylate cyclase to produce cAMP, which activates protein kinase A (PKA). PKA phosphorylates phosphorylase kinase, which then phosphorylates and activates glycogen phosphorylase. PKA also phosphorylates but, in this case, inactivates glycogen synthase.
Gluconeogenesis is stimulated because the synthesis of phosphoenolpyruvate carboxykinase, fructose 1,6-bisphosphatase, and glucose 6-phosphatase is induced and because there is an increased availability of precursors. Fructose 1,6-bisphosphatase is also activated because the levels of its inhibitor, fructose 2,6-bisphosphate, are low (Fig. 34.7). During fasting, the activities of the corresponding enzymes of glycolysis are decreased.
FIGURE 34.7 Regulation of gluconeogenesis (red arrows) and glycolysis (black arrows) during fasting. The gluconeogenic enzymes phosphoenolpyruvate carboxykinase, fructose 1,6-bisphosphatase, and glucose 6-phosphatase are induced (↑). Fructose 1,6-bisphosphatase is also active because, during fasting, the level of its inhibitor, fructose 2,6-bisphosphate, is low. The corresponding enzymes of glycolysis are not very active during fasting. The rate of glucokinase is low because it has a high Km for glucose and the glucose concentration is low. Phosphofructokinase-1 is not very active because the concentration of its activator fructose 2,6-bisphosphate is low. Pyruvate kinase is inactivated by cyclic adenosine monophosphate (cAMP)-mediated phosphorylation. Acetyl CoA, acetyl coenzyme A; F-2,6-BP, fructose 2,6-bisphosphate; Pi, inorganic phosphate.
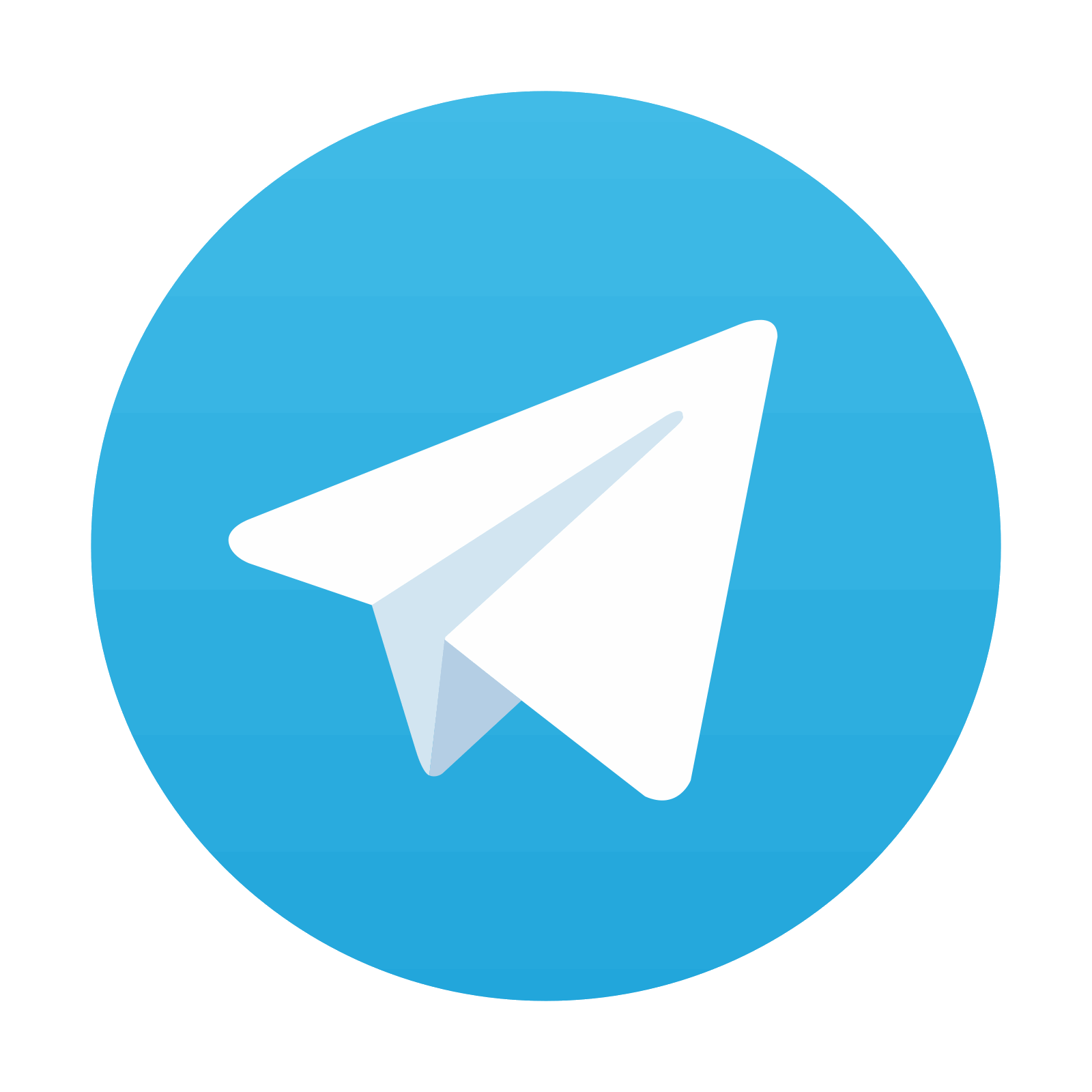
Stay updated, free articles. Join our Telegram channel
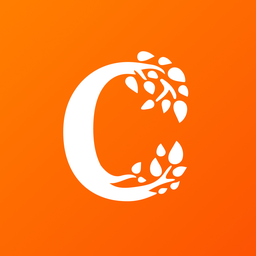
Full access? Get Clinical Tree
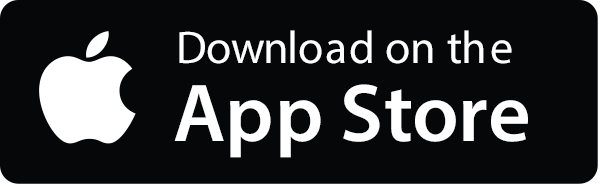
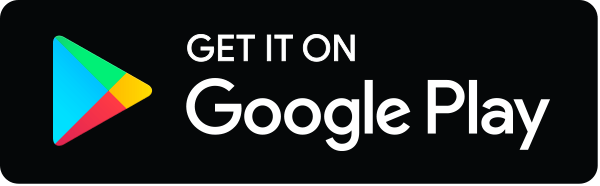