CHAPTER 2 Homeostasis of Body Fluids
Normal cellular function requires that the intracellular composition of ions, small molecules, water, pH, and a host of other substances be maintained within a narrow range. This is accomplished by the transport of many substances and water into and out of the cell with the use of membrane transport proteins as described in Chapter 1. In addition, each day food and water are ingested and waste products are excreted from the body. In a healthy individual this occurs without significant changes in either the volume of body fluids or their composition. Such maintenance of steady-state balance, where the volume and composition of body fluids remain constant despite the addition and elimination of water and solutes from the body, to a large degree reflects the function of epithelial cells. These cells, which constitute the interface between the internal environment of the body and the external world, maintain the volume and composition of the fluid bathing all cells (i.e., the extracellular fluid [ECF]) constant. The ECF, in turn, helps cell maintain a constant intracellular environment.
CONCEPT OF STEADY-STATE BALANCE
In keeping with the dam and lake analogy, consider the maintenance of steady-state water balance in humans (see Chapter 34 for details). Each day various volumes of liquid are ingested, and water is produced through cellular metabolism. Importantly, the amount of water added to the body each day is not constant, although it can be regulated to a degree by the thirst mechanism. In addition, water is lost from the body via respiration, sweating, and feces. The amount of water lost by these routes also varies over time, depending on the respiratory rate, physical activity, ambient temperature, and the presence or absence of diarrhea. The only regulated route for excretion of water from the body is the kidneys. The body maintains steady-state water balance by ensuring that the amount of water added to the body each day is exactly balanced by the amount lost or excreted from the body.
The body monitors the amount of water that it contains through changes in the osmolality of ECF. When excess water is added to the body, the osmolality of ECF decreases. Conversely, when excess water is lost from the body, osmolality increases. Cells within the hypothalamus of the brain monitor changes in ECF osmolality around each person’s genetically determined set point. When deviations from the set point occur, neural and hormonal signals are activated (i.e., effectors). For example, when ECF osmolality is increased, neural signals are sent to another region of the hypothalamus to stimulate the sensation of thirst. At the same time, antidiuretic hormone (ADH) is secreted from the posterior pituitary and acts on the kidneys to reduce the excretion of water. Thus, water intake is increased at the same time that its loss from the body is reduced, and the osmolality of ECF returns to its set point. When the osmolality of ECF is decreased, thirst is inhibited, as is the secretion of ADH. As a result, intake of water is reduced, and its excretion by the kidneys is increased. Again, these actions return the osmolality of ECF to the set point.
OVERVIEW OF THE INTRACELLULAR AND EXTRACELLULAR COMPARTMENTS
Definitions and Volumes of Body Fluid Compartments
As illustrated in Figure 2-1, total body water is distributed between two major compartments, which are divided by the cell membrane.* The intracellular fluid compartment is the larger compartment and contains approximately two thirds of total body water. The remaining third is contained in the extracellular fluid compartment. Expressed as percentages of body weight, the volumes of total body water, ICF, and ECF are
Composition of Body Fluid Compartments
Table 2-1 summarizes the composition of the ECF and ICF for a number of important ions and molecules. As discussed in detail later, the composition of ICF is maintained by the action of various specific membrane transport proteins. Principal among these transporters is Na+,K+-ATPase, which converts the energy in ATP into ion and electrical gradients, which in turn can be used to drive the transport of other ions and molecules.
Table 2-1 Ionic Composition of a Typical Cell
Extracellular Fluid | Intracellular Fluid | |
---|---|---|
Na+ (mEq/L) | 135-147 | 10-15 |
K+ (mEq/L) | 3.5-5.0 | 120-150 |
Cl− (mEq/L) | 95-105 | 20-30 |
HCO3− (mEq/L) | 22-28 | 12-16 |
Ca++ (mmol/L)* | 2.1-2.8 (total) | ≈10−7 (ionized) |
1.1-1.4 (ionized) | ||
Pi (mmol/L)* | 1.0-1.4 (total) | |
0.5-0.7 (ionized) | 0.5-0.7 (ionized) |
* Ca++ and Pi (H2PO4−/HPO4−2) are bound to proteins and other organic molecules. In addition, large amounts of Ca++ can be sequestered within cells. Large amounts of Pi are present in cells as part of organic molecules (e.g., ATP).
The composition of the plasma and interstitial fluid compartments of the ECF is similar because they are separated only by the capillary endothelium, a barrier that is freely permeable to ions and small molecules. The major difference between interstitial fluid and plasma is that the latter contains significantly more protein. Although this differential concentration of protein can affect the distribution of cations and anions between these two compartments by the Gibbs-Donnan effect (see later for details), this effect is small, and the ionic composition of interstitial fluid and plasma can be considered to be identical.
Fluid Exchange between the ICF and ECF
Water moves freely and often rapidly between the various body fluid compartments. Two forces determine this movement: hydrostatic pressure and osmotic pressure. Hydrostatic pressure from pumping of the heart (and the effect of gravity on the column of blood in the vessel) and osmotic pressure exerted by plasma proteins (oncotic pressure) are important determinants of fluid movement across the capillary wall (see Chapter 17). By contrast, because hydrostatic pressure gradients are not present across the cell membrane, only osmotic pressure differences between ICF and ECF cause movement of fluid into and out of cells.
A useful approach to understanding the movement of fluids between the ICF and the ECF is outlined in Figure 2-2. To illustrate this approach, consider what happens when solutions containing various amounts of NaCl are added to the ECF.*
The glucose and urea concentrations are expressed in units of mg/dL (dividing by 18 for glucose and 2.8 for urea* allows conversion from the units of mg/dL to mmol/L and thus to mOsm/kg H2O). This estimation of plasma osmolality is especially useful when dealing with patients who have an elevated plasma [glucose] secondary to diabetes mellitus and in patients with chronic renal failure, whose plasma [urea] is elevated.
Example 1: Addition of Isotonic NaCl to ECF
Addition of an isotonic NaCl solution (e.g., intravenous infusion of 0.9% NaCl, osmolality of ≈290 mOsm/kg H2O)† to ECF increases the volume of this compartment by the volume of fluid administered. Because this fluid has the same osmolality as ECF and therefore also ICF, there will be no driving force for movement of fluid between these compartments, and the volume of ICF will be unchanged. Although Na+ can cross cell membranes, it is effectively restricted to the ECF by the activity of Na+,K+-ATPase, which is present in the plasma membrane of all cells. Therefore, there is no net movement of the infused NaCl into the cells.
MAINTENANCE OF CELLULAR HOMEOSTASIS
Normal cellular function requires that the composition of ICF be tightly controlled. For example, the activity of some enzymes is dependent on pH. Therefore, intracellular pH must be regulated. The intracellular ionic composition is similarly held within a narrow range. This is necessary for establishment of the membrane potential, a cell property especially important for the normal function of excitable cells (e.g., neurons and muscle cells) and for intracellular signaling (e.g., intracellular [Ca++]–see Chapter 3). Finally, the volume of cells must be maintained because shrinking or swelling of cells can lead to cell damage or death. Regulation of intracellular composition and cell volume is accomplished through the activity of specific transporters in the plasma membrane of cells. This section reviews the mechanisms by which cells maintain their intracellular ionic environment and membrane potential and control their volume.
Ionic Composition of Cells
The intracellular ionic composition of cells varies from tissue to tissue. For example, the intracellular composition of neurons is different from that of muscle cells, which differs from that of blood cells. Nevertheless, there are similar patterns, and these are presented in Table 2-1. When compared with ECF, ICF is characterized by a low [Na+] and a high [K+]. This is the result of the activity of Na+,K+-ATPase, which transports 3 Na+ ions out of the cell and 2 K+ ions into the cell for each molecule of ATP hydrolyzed. As will be discussed, the activity of Na+,K+-ATPase is not only important for establishing the cellular Na+ and K+ gradients but is also involved in indirectly determining the cellular gradients for many other ions and molecules. Because Na+,K+-ATPase transports three cations out of the cell in exchange for two cations, it is electrogenic and thus contributes to the establishment of membrane voltage (cell interior negative). However, Na+,K+-ATPase typically contributes only a few millivolts to the membrane potential. More importantly, it is the leakage of K+ out of the cell through K+-selective channels that is a major determinant of membrane voltage (see later). Thus, Na+,K+-ATPase converts the energy in ATP into ion gradients (i.e., Na+ and K+) and a voltage gradient (i.e., membrane potential) as a result of leakage of K+ out of the cell driven by the K+ concentration gradient across the membrane ([K+]i > [K+]o).
The Na+,K+-ATPase–generated ion and electrical gradients are used to drive the transport of other ions and molecules into or out of the cell (Fig. 2-3). For example, as described in Chapter 1, a number of solute carriers couple the transport of Na+ to that of other ions or molecules. The Na+-glucose and Na+–amino acid symporters use the energy in the Na+ electrochemical gradient, directed to bring Na+ into the cell, to drive the secondary active cellular uptake of glucose and amino acids. Similarly, the inwardly directed Na+ gradient drives the secondary active extrusion of H+ from the cell and thus contributes to the maintenance of intracellular pH. The 3Na+-1Ca++ antiporter, along with plasma membrane Ca++-ATPase, extrudes Ca++ from the cell and thus contributes to maintenance of a low intracellular [Ca++].* Finally, the membrane voltage drives Cl− out of the cell through Cl−-selective channels, thus lowering the intracellular concentration below that of the ECF.
< div class='tao-gold-member'>
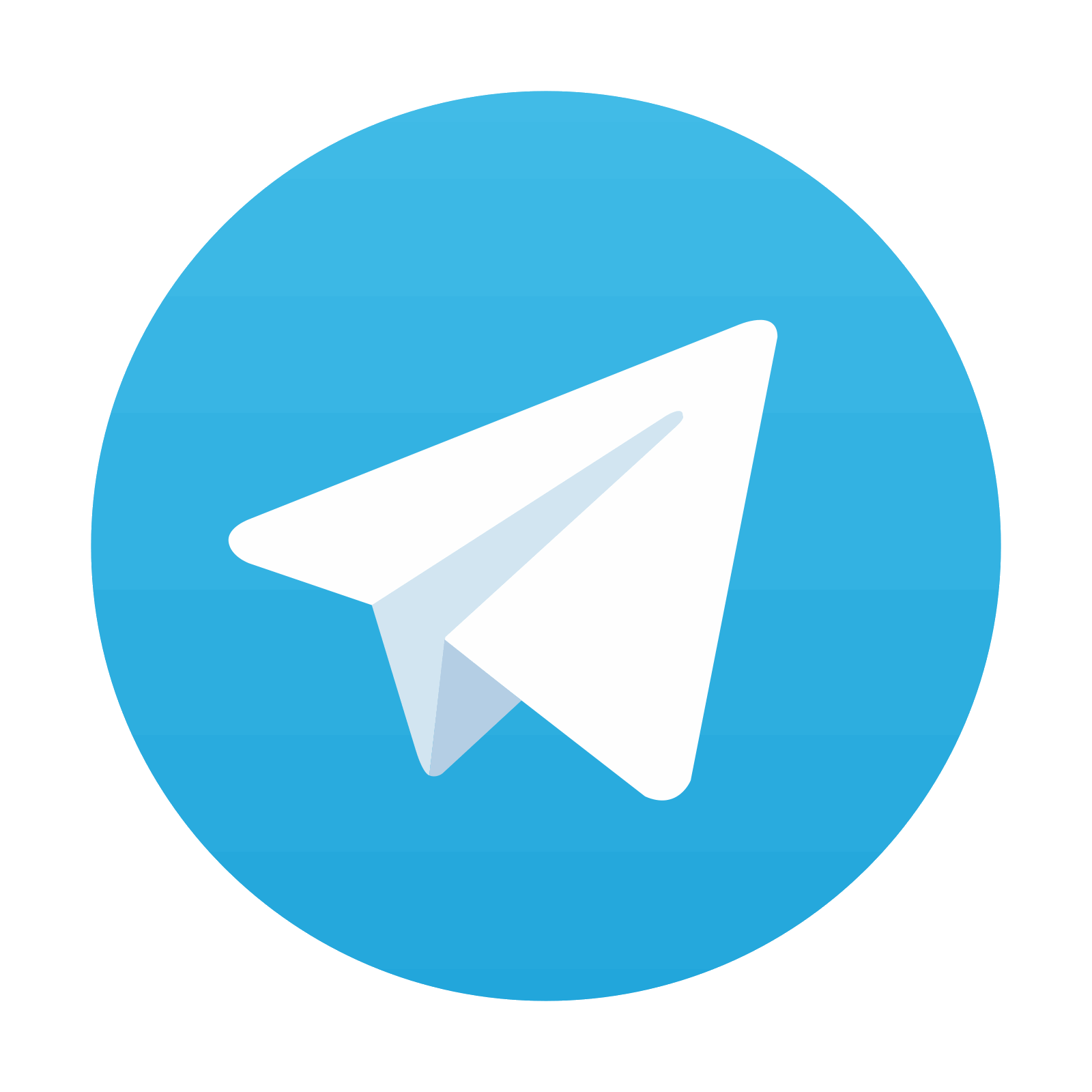
Stay updated, free articles. Join our Telegram channel
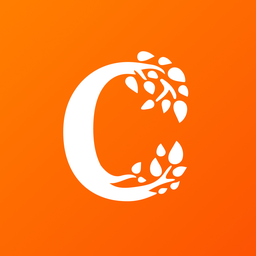
Full access? Get Clinical Tree
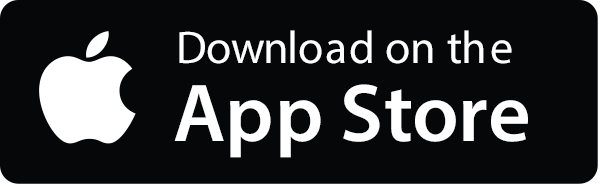
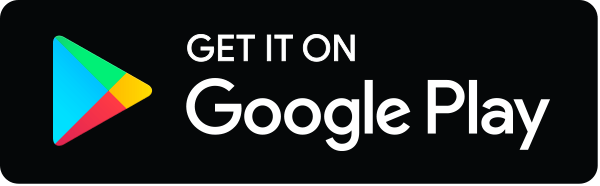