CHAPTER 10 Higher Functions of the Nervous System
Interactions between different parts of the cerebral cortex and between the cerebral cortex and other parts of the brain are responsible for many of the higher functions that characterize humans. The neural basis for some of these higher functions is discussed in this chapter.
THE CEREBRAL CORTEX
The cerebral cortex can be divided into the left and right hemispheres and subdivided into a number of lobes (Fig. 10-1), including the frontal, parietal, temporal, and occipital lobes. These lobes are named for the overlying bones of the skull. The frontal and parietal lobes are separated by the central sulcus; they are separated from the temporal lobe by the lateral fissure. The occipital and parietal lobes are separated (on the medial surface of the hemisphere) by the parietooccipital fissure (Fig. 10-1). Buried within the lateral fissure is another lobe, the insula (Fig. 4-7A). The limbic lobe is formed by the cortex on the medial aspect of the hemisphere that borders on the brainstem. Part of the limbic lobe, the hippocampal formation, is folded into the parahippocampal gyrus of the temporal lobe and cannot be seen from the surface of the brain.
Activity in the two hemispheres of the cerebral cortex is coordinated by interconnections through the cerebral commissures. The bulk of the neocortex on the two sides is connected through the massive corpus callosum (Fig. 10-1). Parts of the temporal lobes connect through the anterior commissure, and the hippocampal formations on the two sides communicate through the hippocampal commissure (which is formed between the fornices on the two sides as they approximate each other at the back of the septum pellucidum and pass under the corpus callosum).
Functions of the Lobes of the Cerebral Cortex
Frontal Lobe
One of the main functions of the frontal lobe is motor behavior. As discussed in Chapter 9, the motor, premotor, cingulate motor, and supplementary motor areas are located in the frontal lobe, as is the frontal eye field. These areas are crucial for planning and executing motor behavior. Broca’s area, essential for the generation of speech, is located in the inferior frontal gyrus of the dominant hemisphere for human language (almost always the left hemisphere, as explained later). In addition, the prefrontal cortex in the rostral part of the frontal lobe plays a major role in personality and emotional behavior.
Parietal Lobe
The parietal lobe contains the somatosensory cortex and the adjacent parietal association cortex (see Chapter 7). This lobe is involved in the processing and perception of sensory information. Connections with the frontal lobe allow somatosensory information to affect voluntary motor activity. Visual information from the occipital lobe reaches the parietal association cortex and the frontal lobe, where it also helps guide voluntary movements. Somatosensory information can also be transferred to language centers, such as Wernicke’s area, in the dominant hemisphere, as described later. The parietal lobe in the nondominant hemisphere is involved in determining spatial context, as shown by the effects of specific lesions (see Chapters 7 and 9).
Occipital Lobe
The primary function of the occipital lobe is visual processing and perception (see Chapter 8). Connections to the frontal eye fields affect eye movements, and a projection to the midbrain assists in the control of convergent eye movements, pupillary constriction, and accommodation, all of which occur when the eyes adjust for near vision.
Temporal Lobe
The temporal lobe has many different functions. One of these functions is hearing, which depends on processing and perception of information related to sounds (see Chapter 8). Another function is processing of vestibular information. Several visual areas have been discovered in the temporal lobe; hence, this lobe is also involved in higher-order visual processing (see Chapter 8). For example, the infratemporal cortex, on its inferior surface, is involved in the recognition of faces. In addition, Meyer’s loop, which forms part of the optic pathway, passes through the temporal lobe. Therefore, temporal lobe lesions can damage vision in part of the visual fields. Similarly, some of Wernicke’s area, essential for the understanding of language, lies in the posterior region of the temporal lobe.
The medial temporal lobe belongs to the limbic system, which participates in emotional behavior and regulates the autonomic nervous system (see Chapter 11). The hippocampal formation is involved in learning and memory (see later).
Neocortical Layering and Subdivisions
The different phylogenetic subdivisions of the cerebral cortex can be recognized on the basis of their layering pattern. The neocortex is generally characterized by the presence of six cortical layers (Fig. 10-2). In contrast, the archicortex has only three layers and the paleocortex has four to five layers.
Cell Types in the Neocortex
A number of different cell types have been described in the neocortex (Fig. 10-2). Pyramidal cells are the most abundant cell type and account for approximately 75% of neocortical neurons. Stellate cells and various other types of nonpyramidal cells make up the balance. Pyramidal cells have a large triangular cell body, a long apical dendrite directed toward the cortical surface, and several basal dendrites. The axon emerges from the cell body opposite the apical dendrite, and those from the larger pyramidal cells project into the subcortical white matter. The axon may give off collaterals as it descends through the cortex. Pyramidal cells use an excitatory amino acid (glutamate or aspartate) as their neurotransmitter. Stellate cells, often called granule cells, are interneurons. They have a small soma and numerous branched dendrites, although many will have an apical dendrite and thus look like small pyramidal cells. Some are excitatory interneurons; these cells are abundant in layer IV of the cortex (see below). Their axons remain in the same cortical region and frequently ascend into the upper cortical layers. Other stellate cells are inhibitory interneurons that use γ-aminobutyric acid (GABA) as their neurotransmitter.
Cytoarchitecture of Cortical Layers
Each of the six layers of the neocortex has a characteristic cellular content (Fig. 10-2). Layer I (molecular layer) has few neuronal cell bodies; instead, it contains mostly axon terminals and synapses on dendrites. Layer II (external granular layer) contains mostly stellate cells. Layer III (external pyramidal layer) consists mostly of small pyramidal cells. Layer IV (internal granular layer) contains mostly stellate cells, including the excitatory type. Layer V (internal pyramidal layer) is dominated by large pyramidal cells. These cells are the main source of cortical efferents to most subcortical regions. Layer VI (multiform layer) contains pyramidal, fusiform, and other types of cells. This layer is also an important origin of cortical efferents, those that target thalamic nuclei.
Cortical Afferent and Efferent Fibers
The cortical efferent axons originate from pyramidal cells. The pyramidal cells of layers II and III project to other cortical areas, either ipsilaterally or contralaterally, via the corpus callosum. The pyramidal cells of layer V project in many descending pathways and have synaptic targets in the spinal cord, brainstem, striatum, and thalamus. The pyramidal cells of layer VI form corticothalamic projections to the thalamic nuclei with specific cortical projections. Reciprocal thalamocortical and corticothalamic interconnections are likely to make important contributions to the electroencephalogram (EEG) (see later).
Regional Variations in Neocortical Structure
On the basis of differences in cytoarchitecture, a number of subdivisions of the neocortex can be recognized. Most of the cortex is composed of six readily distinguishable layers. The primary and premotor areas are sometimes said to be agranular cortex. This is a misnomer because all cortical areas, including these motor areas, have similar percentages of pyramidal and nonpyramidal cells (≈75% versus 25%). However, in the frontal motor areas, the nonpyramidal cell bodies do not group in a manner that leads to the formation of distinct “granular” layers. Moreover, local inhibitory interneurons play an important role in somatotopic organization of the primary motor cortex (see Chapter 9).
Another type of cortex has a very prominent layer IV and thus is called granular cortex. Dominated as it is by the stellate cells seen in layer IV of Figure 10-2, it is specialized for processing afferent input. Therefore, this kind of cortex is found in the primary sensory receiving areas: the somatosensory cortex (S-I), the primary auditory cortex, and the primary visual (striate) cortex. The striate cortex is given its name because of a particularly prominent horizontal sheet of axons in layer IV known as the stripe of Gennari.
Most of the other regions of cortex show less dramatic variations and often seem to grade from one type of layer morphology to the next as one looks at adjacent areas of cortex. On the basis of such an extensive cytoarchitectural analysis, Brodmann divided the cortex into 52 discrete areas (Fig. 10-3). Commonly referred to areas include Brodmann’s areas 3, 1, and 2 (the S-I cortex of the postcentral gyrus); area 4 (the primary motor cortex of the precentral gyrus); area 6 (the premotor and supplementary motor cortex); areas 41 and 42 (the primary auditory cortex on the superior temporal gyrus); and area 17 (the primary visual cortex mostly on the medial surface of the occipital lobe). Detailed studies have confirmed that the Brodmann areas are, in fact, distinctly different, both with respect to their interconnections and with respect to their functions, but more recent work has shown that there is some plasticity, both in the size of the areas and in their internal organization (see later).
Archicortex and Paleocortex
Hippocampal Formation
In humans the hippocampal formation is part of the archicortex. It is folded into the temporal lobe and can be viewed only when the brain is dissected. The hippocampal formation consists of several parts, including the hippocampus (Ammon’s horn or cornu ammonis), the dentate gyrus, and the subiculum. These divisions are well demarcated in a cross section through the hippocampal formation (Fig. 10-4).
The hippocampus has three layers: the molecular, pyramidal cell, and polymorphic layers. They resemble layers I, V, and VI in the neocortex. The folding of the hippocampus imparts an inverted appearance because the white matter is at the surface of the lateral ventricle (Fig. 10-4). The white matter covering the hippocampus is called the alveus, which contains hippocampal afferent and efferent fibers. The axons in the alveus continue into a nerve fiber bundle called the fimbria; the fimbria is continuous with the fornix.
The hippocampal formation receives its main neural input from the entorhinal cortex of the parahippocampal gyrus. Important, generally reciprocal connections are formed between the pyramidal cells of the hippocampus and (1) the septal nuclei and mammillary body by way of the fornix and (2) the contralateral hippocampal formation by way of the fornix and the hippocampal commissure. The hippocampus is a major component of Papez’s circuit (see Fig. 11-6).
HIGHER FUNCTIONS OF THE NERVOUS SYSTEM
The Electroencephalogram
An EEG is a recording of neuronal electrical activity that can be made from the cerebral cortex via electrodes placed on the skull. In an electrocorticogram, electrical activity of the cortex is recorded via electrodes placed on the surface of the brain. These are both called field potentials because they detect the electrical field generated by large groups of relatively distant neurons. The EEG waves are derived from the excitatory and inhibitory synaptic potentials that occur in cortical neurons as a result of thalamocortical and other input, and they are produced chiefly by extracellular currents that flow across the cortex during the generation of synaptic potentials in the pyramidal cells. The potentials recorded as the EEG are relatively large (around 100 μV) and reflect the activity of many pyramidal cells, which are arranged with their apical dendrites aligned in parallel to form a dipole sheet. One pole of this sheet is oriented toward the cortical surface and the other toward the subcortical white matter. Note that the sign of an EEG wave does not in itself indicate whether pyramidal cells are being excited or inhibited. For instance, a negative EEG potential may be generated at the surface of the skull (or cortex) by excitation of apical dendrites or by inhibition near the somas. Conversely, a positive EEG wave can be produced by inhibition of apical dendrites or by excitation near the somas. Although a brief EEG wave is sometimes referred to as a spike, this term does not refer to action potentials because the extracellular currents associated with action potentials are too small, fast, and asynchronous to be recorded with EEG electrodes.
A normal EEG consists of waves of various frequencies. The dominant frequencies depend on several factors, including the state of wakefulness, the age of the subject, the location of the recording electrodes, and the absence or presence of drugs or disease. When a normal awake adult is relaxed with the eyes closed, the dominant frequencies of the EEG recorded over the parietal and occipital lobes are about 8 to 13 Hz, the alpha rhythm. If the subject is asked to open his eyes, the EEG becomes less synchronized and the dominant frequency increases to 13 to 30 Hz, which is called the beta rhythm. The delta (0.5 to 4 Hz) and theta (4 to 7 Hz) rhythms are observed during sleep (see the following discussion) (Fig. 10-5).
< div class='tao-gold-member'>
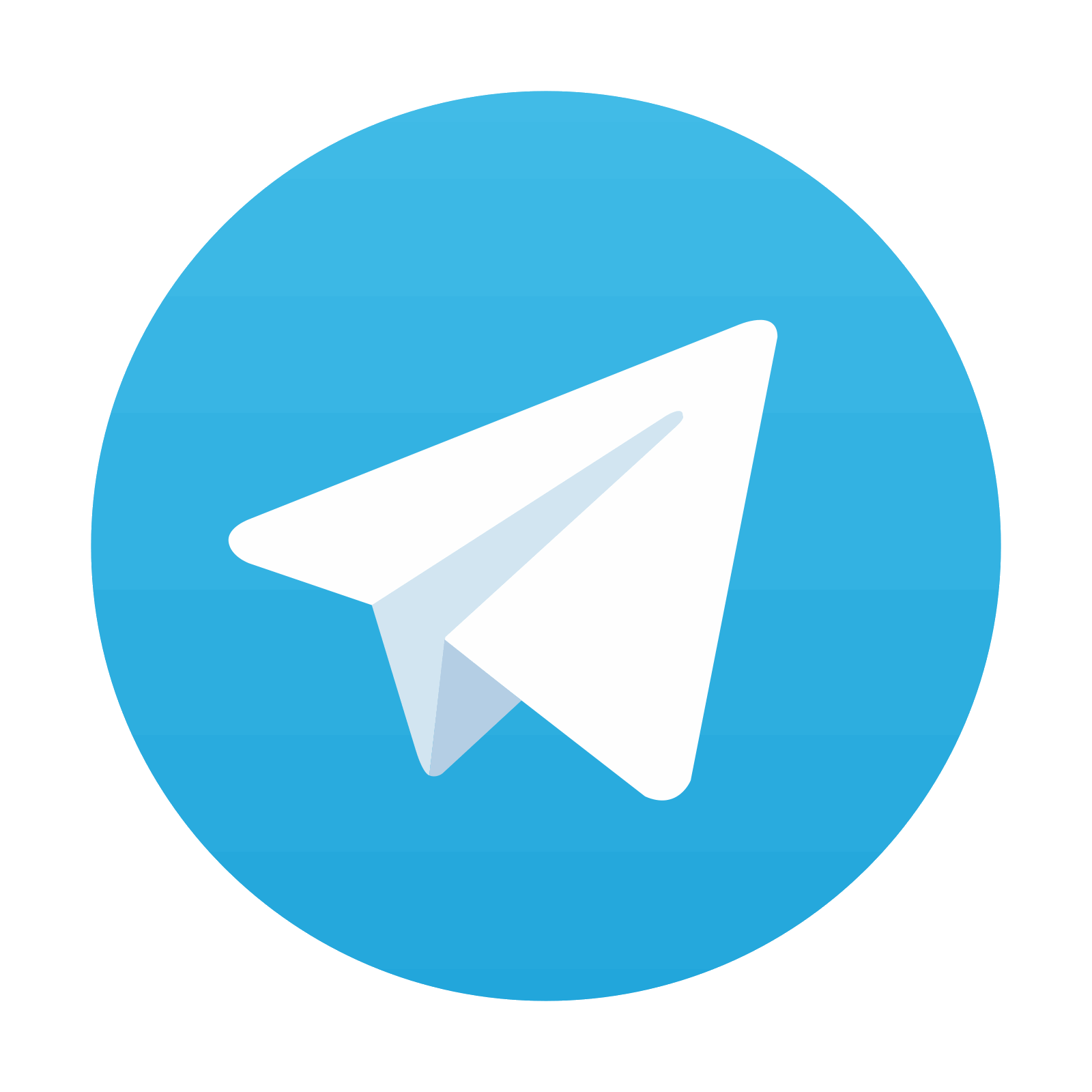
Stay updated, free articles. Join our Telegram channel
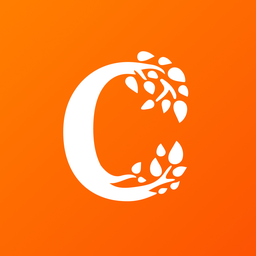
Full access? Get Clinical Tree
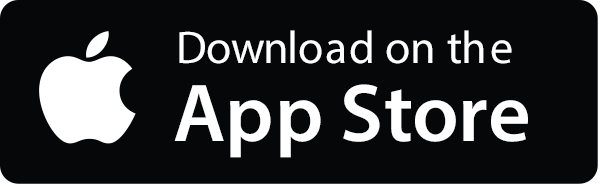
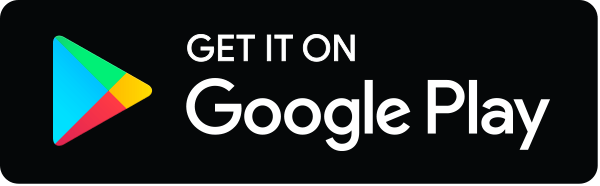