A. OVERVIEW
The technology of glass and ceramics is one of the oldest human activities. The fabrication of food and liquid storage containers from clay and glass, and the production of arrowheads and other weapons from flint and other minerals, marks the beginning of technological skill in the human race. Glass and ceramics remain an important component of technology today, and large numbers of the applications on which we depend for modern living utilize ceramics in one form or another. For the most part, ceramics are totally inorganic materials, typically high-melting, often heavily crosslinked, and relatively intractable once they are fabricated.
In contrast to classical chemistry, mineralogical and ceramics science uses a special way to describe molecular and materials structures. It is based on the concept of connected tetrahedra, octahedra, and other structures through edges or corners rather than by drawing specific bonds between the participating elements. It allows a visual simplification of structures without loss of detailed information. The method works as follows. Elements such as silicon, which use four tetrahedrally oriented bonds to connect to other atoms, are represented by the center of a tetrahedron, with the four atoms to which the silicon is connected represented by the corners of the tetrahedron (Figure 7.1). Thus, rings and chains of alternating silicon and oxygen atoms can be depicted as a series of tetrahedra connected through their corners, with the remaining two corners at each silicon being the location of side groups attached to silicon (see the examples in Figures 7.2 and 7.3). Ladder silicate structures are then represented by two chains of tetrahedra connected through corners (Figure 7.4). Sheet structures or three-dimensional arrangements are shown as tetrahedra connected through edges i.e., utilizing two oxygens linked to each silicon). Elements such as aluminum, which can occupy sites in a solid structure connected to six oxygen atoms, are depicted at the center of an octahedron, with connections to adjacent octahedra or tetrahedra being through corners or edges.
Figure 7.1. The tetrahedral orientation of bonds to silicon, and the use of a tetrahedron as a building block in the depiction of silicate structures.

Figure 7.2. Illustration of the use of tetrahedra to describe cyclic metasilicate molecules. (From Greenwood, N. N.; Earnshaw, A., Chemistry of the Elements , Pergamon Press, 1984. Reproduced with permission.)

Ceramics and glasses can be classified into three types: (1) oxide-type materials that are produced from mineralogical materials by means of relatively simple chemical processes or no chemical modification at all, (2) oxide ceramics that resemble their mineralogical counterparts but are synthesized and fabricated from small-molecule precursors via low- or moderate-temperature processes, and (3) nonoxide ceramics—materials that only rarely exist in nature but are produced by high-temperature chemical processes either from the elements or from polymeric precursors. These three types of materials are discussed in turn.
Figure 7.3. The structures of various metasilicate polymer chains depicted in terms of the number of tetrahedral silicate units per repeat distance along each chain. The repeat distances are given in picometers.

B. OXIDE CERAMICS AND GLASSES OBTAINED OR PRODUCED DIRECTLY FROM MINERALOGICAL MATERIALS
1. General Observations
Oxide ceramics and glasses are the classical materials that are the basis of a large part of traditional technology. They include various forms of glass, objects derived from clays such as brick and fine china, mica furnace windows, alumina and titania insulators, and the minerals used in buildings such as limestone, granite, sandstone, and concrete blocks,
Figure 7.4. Ladder silicates depicted in terms of silicate tetrahedra. (From Wells, A. F., Structural Inorganic Chemistry , 4th ed. Oxford University Press, 1975, p. 816. Reproduced with permission.)

2. Silica, Silicates, and Aluminosilicates—General Characteristics
Oxygen and silicon are the two most abundant elements in the earth’s crust. Consequently, an enormous number of different forms of silicon–oxygen-based minerals exist, estimated to number more than 25% of all known minerals, and these represent more than 90% of the earth’s crust. Yet, despite this diversity, there is only one building block for all the multiplicity of known silicates—the tetrahedral arrangement of four oxygens linked to a central silicon. In nearly all of these tetrahedra the Si—O distance is close to 1.6 Å and the O…O distances at the boundaries of each tetrahedron are near 2.7 Å. Depending on the ratio of Si—O–Si linkages to the number of charged Si—O-units, cations are also present to balance the charges. Note that aluminum (Al3+) often becomes incorporated into a silicate lattice to give aluminosilicates. When this happens, changes in the cation numbers are required to balance the charges.
Silicates are classified into six related structural groups that are easily understood in terms of the tetrahedral silicate building block:
1. Those species with a single-silicate tetrahedron, , associated with cations such as Na+, K+, and Mg2+. An example structure is tetrasodium silicate, depicted schematically in Figure 7.5.
2. Short chains or small rings of tetrahedra linked through corners, with cations to balance the charges of those oxygen atoms not involved in the chain or ring structure. Examples are shown in Figure 7.2.
3. Long, single-stranded or double stranded (ladder) polymer chains that consist of tetrahedra, again linked through corners (Figure 7.3). Single-stranded polymers are known as pyroxenes. Ladder or ribbon polymers (Figure 7.4) are called amphiboles, an example of which is chrysotile (white) asbestos: Mg2Si2O5(OH)4.
4. Sheet structures are formed by connections between three of the four corners of each tetrahedron; the remaining corner oxygen atoms is associated with charge-balancing cations (Figure 7.6). Many examples exist of sheet structures that form multilayer sandwiches separated by galleries that contain the cations and sometimes water molecules. This type of structure is found in many clays, mica, and vermiculite. If those cations are divalent, such as calcium or magnesium, the layers may be connected by ionic crosslinks. If the cations are monovalent, the layers will not be connected and can often be separated under suitable conditions. Some minerals have pendent hydroxyl groups that do not condense, either because the solid-state structure provides too great a separation or because the interlayer regions contain trapped water. These layers can often be separated easily.
5. Cuboid-type nanostructures in which eight silicon atoms exist at the corners of a cube, connected through Si—O–Si bonds. These are called “sesquisiloxanes.” Most of these species are produced in the laboratory, but they can be visualized as intermediates in the mineralogical formation of more extensive structures.
6. Ceramics in which all four corners of the silicate tetrahedra are connected to other tetrahedra through Si—O–Si linkages to give a three-dimensional, charge-neutral framework. Silica (SiO2), often found in the form of sand, falls into this category. Crystalline silica exists in three different modifications—quartz, tridymite, and crystobalite. All three exist with two different modifications (α and β), and have different melting points and slightly different arrangements of the tetrahedra in the lattice. However, quartz is by the far the most common variety. The structure of christobalite is shown in Figure 7.7.
Figure 7.5. A monosilicate with all four oxygen atoms bearing anionic charges. Such species, with no Si—OH units, are unable to undergo condensation to rings, chains, or three-dimensional ultrastructures.

Figure 7.6. Sheet structures formed by extended two-dimensional condensation of silicate hydroxyl groups. Sheet structures are favored if one of the four oxygen atoms per silicon atom is in the form of a salt [i.e., (HO)3SiO−M+]. (a) View at right angles to the sheet structure; (b) view along a sheet.

Another way to visualize the structure of silicates is as species formed when some of the Si—O–Si connections in silica are broken by an aqueous base such as sodium hydroxide. As these connections are progressively broken, the system becomes decreasingly crosslinked and changes from a three-dimensional network into a system of chains and rings (Figure 7.8).
Figure 7.7. The covalently linked, three-dimensional atomic arrangement in cristobalite (SiO2). (From Kingery, W. D., Bowen, H. K., and Uhlmann, D. R., Introduction to Ceramics, 2nd ed., Wiley, 1976.)

Still another way to understand silicate structures is as polymers formed by the condensation of Si—OH units derived from silicic acid, Si(OH)4. From the discussion in Chapter 6, it will be clear that the products formed by condensation will depend on the number of functional OH groups attached to silicon. Hydroxyl groups that have been converted to Si—O−M+ units are essentially unreactive and will remain as side groups to the chains, rings, sheets, or ultrastuctures formed during condensation. For example, condensation of the difunctional (NaO)2Si(OH)2 will give a linear silicate polymer of the type shown in Figure 7.3. Alternatively, condensation of NaOSi(OH)3 can yield ribbon or ladder polymers or sheets of rings, as shown in Figure 7.4, with the Si—O−Na+ groups oriented above or below the plane of the fused rings It follows that, if the sheet structure bears pendent OH rather than O–Na+ groups, the sheets themselves can be joined by condensation to form a three-dimensional structure like silica. If a template unit such as water or large ions are present, these may cause the framework to grow around them and give rise to tunnels or cavities.
Figure 7.8. Conversion of silica glass to a lower-melting-point soda glass through cleavage of Si—O bonds by sodium hydroxide or sodium carbonate. (From Thrower, R. A., Materials in Today’s World 2nd ed. McGraw-Hill, 1996.)

An additional level of complexity is reached when aluminum atoms are incorporated into the structure in place of some of the silicon atoms to give aluminosilicates. Because aluminum hydroxide contains only three hydroxyl groups rather than the four of silicic acid, the normal condensed silicate tetrahedral arrangement becomes interrupted. This has far-reaching consequences, including the formation of discontinuities in both structure and charge neutralization. Moreover, aluminum can form six coordinate bonds to oxygen, and often does so in three-dimensional lattices. In this way the presence of aluminum in a silicate lattice leads to the formation of layered structures in which the galleries between the layers are occupied by water; monovalent cations, to maintain charge neutrality; or divalent cations, which ionically bind the layers together but that can be disrupted by ionic reagents. Zeolites are aluminosilicate variants of these structures in the sense that, instead of layers, they contain cavities or tunnels in which ions, water, or other small molecules can be accommodated. They are discussed later in this chapter.
3. Aluminosilicate Clays and Related Minerals—Properties and Structure
Clay minerals are finely divided particulate materials found in almost every region of the globe. They are an essential component of soil. The most familiar clays are layered aluminosilicate materials such as china clay (kaolinite) and fuller’s earth (montmorillonite or bentonite). Vermiculite and mica are layered aluminosilicate minerals that do not form clays but are found in igneous rocks. Talc is a closely related layered material formed from a silicate rather than an aluminosilicate.
Kaolinite is one of the main materials used for the manufacture of china clay ceramics. It is also a key component in the manufacture of high-quality paper since it provides gloss and body to what would otherwise be a highly porous product. Prodigious amounts of these minerals are mined and distributed each year; the main sources are in the United States, United Kingdom, Russia, and China.
Clays have an aluminosilicate sandwich structure based on laterally connected silicate tetrahedra connected (crosslinked) to a layer of laterally connected alumina octahedra. There may be just two layers per sandwich unit—one silicate and one aluminate, or three layers—one aluminate flanked by two silicate or vice versa. The silica and alumina layers are connected covalently through oxygen atoms, but the two- or three-layer plates are stacked together, separated by “galleries.” Each aluminum atom is connected to six neighboring oxygen atoms (rather than the expected three) because the coordination sphere of aluminum can readily accommodate six oxygen donor units. Charge balancing usually requires that cations also be present in the lattice, usually in the galleries that separate the covalently bonded layers. These cations can be hydrogen ions, alkali metal cations, or multivalent cations such as Ca2+ or Mg2+. Mutivalent cations in the galleries may hold the layers together firmly by ionic crosslinking. The size of the cations determines the thickness of the galleries. One type of cation can be replaced by another.
These minerals fall into two different structural categories—those with double-layer plates separated by galleries (bilayer systems), and those with triple-layer plates also separated by galleries. Water molecules or cations occupy the galleries. A schematic representation of a bilayer arrangement is shown in Figure 7.9, and an example of this structure in the form of the mineral Ca2Al2SiO8 is shown in Figure 7.10. Schematic representations of some typical trilayer systems are shown in Figure 7.11., and the building block atomic arrangements for representative systems are shown in Figure 7.12.
Those systems with weak forces between the plates, specifically, with monovalent cations or water in the galleries, often allow the layer plates to separate in liquids to form colloids. For example, the presence of monovalent sodium or hydrogen ions in the galleries can lead to inclusion of water and partial or complete separation of the layers. This may be one reason why the character of the clay in a garden soil or in an artist’s studio undergoes a dramatic change in properties if the pH is altered. Separation of the plates is also facilitated when the original inorganic cations are displaced by large organic cations such as tetrabutylammonium ions. The ceramic may then disintegrate into individual plates that form as colloids in liquid media. A change of pH or the presence of another cation can then induce restacking of the plates, but not always in the original pattern. For example, although it is possible for the plates to restack in a face-to-face structure, it is also possible for them to form edge-to edge aggregates or “house of cards” arrangements. Widening of the galleries by tetraalkylammonium ions also facilitates entry of water-soluble organic or inorganic polymers into the gallery space, a change that can strengthen the materials’ properties.
Figure 7.9. Schematic representation of a two-layer galleried silicate or aluminosilicate. The galleries contain cations or water. If the cations are di- or trivalent, the layer-to-cation attractions will stabilize the three-dimensional structure. If the cations are monovalent, such as Na+, K+, or H+, the double-layer sheets may be induced to separate them from their neighbors as water or other small molecules penetrate the galleries and ease the sheets apart. Also shown is a representation of the structure of Ca2Al2Si2O8.

Figure 7.10. (a) View along the double-layer aluminosilicate sheets in held together by oxygen crosslinks (open circles). The sites marked with closed circles are occupied by equal numbers of aluminum and silicon atoms. (b) Structure formed by the insertion of 6-coordinated Ca2+ ions into the galleries between the double layers. (Modified from Greenwood, N. N.; Earnshaw, A., Chemistry of the Elements , Pergamon Press, 1984.)

Figure 7.11. Schematic view along the sheets of three-layer galleried silicates and aluminates. The variations in structure and properties of different minerals arise from the different elemental compositions of each of the three layers and the nature of the molecules or ions in the galleries. In all cases the three layers in each assembly are held together by oxygen crosslinks.

Figure 7.12. (a) Aluminosilicate building blocks found in common clay-type minerals; (b) organization of these units in muscovite mica [K2Al4(Si6Al2)O20(OH)4], hydrated montmorillonite [Al4Si8O20(OH)4x H2O], and chlorite [Mg10Al2(Si6Al2)O20(OH)16] galleried structures. (From Greenwood, N. N.; Earnshaw, A., Chemistry of the Elements , Pergamon Press, 1984. Reproduced with permission.)

“Pillared clays” are galleried clays with more permanent structures that separate the layers. An example is the structure formed by replacement of the original cations in the galleries by large aluminate cations. Steps are first taken to ensure that the aluminate pillars are uniformly distributed throughout the galleries. Then the face-to-face galleried ceramic is heated to “fix” each pillar in place as a permanent feature. The final structure can be viewed as resembling a multilevel parking garage with pillars in fixed positions on each level, or as a coal mine in which the “pit props” support the roof of each gallery. The size of the pillars defines the height of the galleries. Once formed, a galleried clay can absorb other molecules into the galleries, and the height of the gallery and the number of pillars per unit area control the size of the molecules that can enter or leave. Thus, galleried clays may be employed to separate small molecules in much the same way as in zeolites. Moreover, polymer molecules can be intercalated into the galleries and used to strengthen the ceramic or to provide some other property such as ionic conductivity. β” Alumina is a pillared ceramic that has no silicate component.
Kaolinite clay is the basis of a very large industry. Its three-layer structure is shown schematically in Figure 7.11, and the basic building block is depicted in Figure 7.12. The structure can be visualized as a stack of three-layer plates with each plate consisting of one layer of sheet silicate sandwiched between and covalently bonded on both sides through oxygen bridges to a layer of sheet aluminate. The exposed aluminate layers bear Al—OH units that point into the galleries between the plates. The repeating distance normal to the sheets is 7.15 Å. The trilayer plates in kaolinite are apparently held together by weak van der Waals and hydrogen bonding forces, and they can be separated fairly easily by the absorption of water. The rheology of kaolinite clay depends on pH, presumably because of the amphoteric (acidic or basic) nature of Al—OH groups and its influence on water absorption and layer displacement.
Montmorillonite clays have been studied in great detail. They have the formula [M2Si4O10(OH)2]−0.330.33 Na+ xH2O, where M = Al1.67 and Mg0.33. This class includes several minerals that share a general structure but differ in minor ways. The basic structure is shown schematically in Figures 7.11 and 7.12. Montmorillonite itself has a three-layer lamellar structure separated by galleries. Each plate within the structure consists of two layers of silicate sheets bonded to each other via oxygen links to a sandwiched layer of aluminum and/or magnesium atoms. The trilayer plates are separated from their neighbors by galleries that contain protons or monovalent cations, together with water molecules. The repeat distance from stack to stack is about 15 Å. Because any “free” hydroxyl units are in the middle of each sandwich rather than on the surface, there are no Al—O−M+ or Si—O−M+ groups facing the galleries to cause ionic crosslinking. This is a difference from the structure of kaolinite. Thus, one type of cation in the galleries is readily replaced by another, and trapped water molecules may be removed by heating and replaced by hydration. Moreover, the water is readily replaced by hydrophilic organic solvents and even by hydrophilic polymers.
Fuller’s earth is a close relative of montmorillonite in which Al—O−H+ units within the lamellar structure are charge-balanced by Ca2+ ions in the galleries. The ability of divalent cations to form ionic crosslinks reduces the tendency of this material to undergo lamellar separation. Fuller’s earth is used for the removal of impurities from liquids during filtration, presumably by ion exchange within the galleries.
Bentonite is the species in which Al—OH protons in the central layers of montmorillonite have been replaced by sodium ions, which occupy the galleries. Bentonite is used in large quantities as a drilling mud. Because the bentonite lamellae bear monovalent cations on their surface, this mineral absorbs substantial quantities of water into the galleries, which causes separation of the plates to form a colloidal dispersion. This process yields very small colloidal particles with negative charges on the flat surfaces and positive charges on the edges. The attractive forces between these oppositely charged components generates a thixotropic gel that liquefies when the colloid is sheared and gels again when the shear forces cease. This is a valuable property in oil drilling.
Pyrophillite [Al2Si4O10(OH)2] can be viewed as a parent structure from which the three-layer aluminosilicate sandwich minerals are derived (Figure 7.12). Its structure consists of a central aluminate layer covalently bonded above and below to silicate layers. The Al—OH groups are buried within the aluminate layer and are therefore less accessible for replacement of protons by metallic cations.
Vermiculite is a magnesium aluminosilicate material that is widely used as a nonflammable packing substance, especially valuable for its ability to absorb liquids from broken bottles and other sources. Vermiculites are produced by the thermal dehydration of various montmorillonite structures that contain magnesium. The dehydration process causes the mineral to form strange, porous, lightweight, worm-like shapes, hence the name vermiculite. An idealized sandwich structure is summarized in Figure 7.11, in which each plate consists of a condensed magnesium hydroxide layer flanked above and below by aluminosilicate layers. The galleries contain water and Mg2+ crosslinking cations. The absorptive powers of this material are due to both the porosity and the presence of galleries.
Micas (also known as muscovite or illite) are transparent aluminosilicate minerals that can be readily separated into the thin sheets and used in the windows of high-temperature equipment (furnaces, home heaters, etc.). The solid-state structure (Figure 7.11) consists of a lamellar arrangement with potassium, calcium, or H3O+ ions occupying the galleries. Micas can be visualized as being formed by replacement of one-quarter of the silicon atoms in pyrophyllite by aluminum. The requirements for charge balancing then lead to the need for different cations in the galleries. The presence of aluminum or magnesium hydroxide cations in the galleries yields another mineral known as chlorite (Figure 7.12).
Talc is a three-layer potassium magnesium silicate rather than an aluminosilicate. Its structure is shown schematically in Figure 7.11. The galleries are occupied by potassium ions, which cannot crosslink the layers. Thus, the magnesium silicate layers can slide past each other. Hence the use of this material in talcum powder.
4. Chrysotile and Other Forms of Asbestos
Asbestos is a general name for several fibrous minerals that were once widely used as nonflammable thermal insulation materials in buildings, ships, brake linings, floor and roof tiles, and electrical wiring. However, the health hazards associated with the inhalation of asbestos fibers have caused severe restrictions on the use of these materials and have prompted widespread efforts to remove asbestos from existing buildings. The health hazards are believed to be a consequence of the narrow diameter (~1 μm) and twisted morphology of the fibers, which favors their penetration into and retention in lung tissue.
Three different forms of asbestos have been used: white asbestos (chrysotile), brown asbestos (amosite), and blue asbestos (crocidolite). Of these, blue asbestos is believed to be the most dangerous form. All three are found as fibrous deposits in metamorphic formations, and they were probably generated by crystallization of the mineral from molten rock. White asbestos is the form most widely used in the United States. This is believed to be less hazardous than the blue form but is nevertheless a suspected carcinogen. Asbestos fibers can be readily distinguished from glass fibers when rotated between crossed polarizers on a microscope stage. Asbestos fibers show bright birefringent colors because they are crystalline, whereas glass, which is amorphous, does not.
Figure 7.13. Chrysotile asbestos is a bilayer system with silicate and magnesium oxide/hydroxide layers. The greater surface area required by the magnesium oxide/hydroxide layer causes each plate to curve into a spiral straw arrangement.

Chrysotile is a member of a large class of minerals known as serpentines, which have the general formula (Mg or Fe)3Si2O5(OH)4. Chrysotile asbestos is a two-layer fibrous magnesium silicate with the formula Mg3(Si2O5)(OH)4, and with the structure shown schematically in Figure 7.13. Thus, it resembles the layered arrangement of the clay-type species described in the preceding sections, but with magnesium oxide units on one side only of each plane. This causes each layered plane to bend away from the magnesium side to form coiled ribbons—ribbons that have wound inward to form a straw-like structure as shown in Figure 7.13.
Amosite is a dark-colored iron silicate with a composition such as Fe7Si8O22(OH)2, which is a member of a group of minerals known as amphiboles (not all of which are fibrous). These species are ladder-type structures that contain two linear silicate chains connected through every fourth repeating unit by oxygen atoms that lie at the corners of the silicate tetrahedra. Thus, the structure of amosite can also be viewed as ribbons of connected 16-membered rings. Crocidolite is another colored amphibole, with the formula Na2Fe5Si8O22(OH)2. The structure is believed to be similar to that of amosite.
5. Glasses
a. General Features.
Glasses are amorphous solids that are usually transparent. Useful glasses have glass transition temperatures (Tg) well above room temperature. A few types of glass are derived from organic polymers, but the traditional inorganic glasses are produced mainly from molten oxides and their salts, such as silica, silicates, borates, or phosphates, many of which have been modified to disrupt crystallinity.
b. Methods of Glass Formation.
Glasses are formed in several different ways. First, a crystalline material such as quartz may be heated above its melting point (~2000°C) and then cooled rapidly (“quenched”) to form a glass. Note that volcanic eruptions often provide the conditions for glass formation on a massive scale. A glass formed by quenching can be considered as a supercooled liquid that could, in theory, crystallize over a long period of time, but is prevented from doing so by the high viscosity of the system at room temperature. This method of glass formation is based on the principle that the ordered state of the crystalline phase is lost on melting, and the randomness of the liquid state is frozen into the glassy material when the system is quenched. Many other crystalline inorganic (and organic) compounds can be induced to form glasses by the same technique. The ease with which the amorphous glass reverts to the crystalline state depends on the ease of molecular movement in the glassy state. Large, unsymmetric or hydrogen-bonded molecules are less likely to revert than small, symmetric species. In the case of quartz, it seems likely that melting disrupts the material into chains or rings but not down to the level of individual tetrahedra.
A special form of silica glass is known as a glass ceramic or Pyroceram (Corning Ware®), which is prized for its resistance to impact and thermal shock. This material is opaque rather than transparent because it consists of fused silica that has been induced to crystallize so that only 10% remains as amorphous glass and 90% consists of silica microcrystals. This conversion from glass to glass ceramic is accomplished by seeding the molten silica before fabrication and solidification with small crystals of titanium dioxide to nucleate the growth of silica crystals within the glass. Controlled heating of the solid glass object then induces growth of silica microcrystals from the amorphous matrix. In the final product the remaining glass serves as a strong binder between the crystals, while the crystallites interrupt crack formation and increase the density of the material. The coefficient of thermal expansion is also reduced, and this, in turn, increases the thermal shock resistance.
A second type of glass is formed by deliberately disrupting the bonds that hold a supermolecular crystalline material together. Quartz is crystalline silica with a regular, three-dimensional repeating arrangement of silicon atoms bonded tetrahedrally to four oxygens throughout the lattice. The addition of reagents that break silicon–oxygen bonds at elevated temperatures, such as metal oxides or carbonates, disrupts the three-dimensional lattice and converts quartz into a random mixture of silicate chains and rings (see Figure 7.8). This creates solid state disorder, lowers the melting point, and inhibits or prevents crystallization. Ordinary window glass, or bottle glass is produced in this way by the addition of sodium or potassium carbonate and calcium oxide.
Note that, as discussed in Chapter 6, a third type of glass is formed from linear or branched polymers. Unless the polymer molecules are highly symmetric, it is difficult for the chains to pack together in crystallites. The same situation exists with totally inorganic or hybrid polymers such as linear polysilicates, polyphosphates, or polyborates, as well as some high-Tg polyphosphazenes.
c. Silicate Glasses.
Silicate glasses are by far the best known, and have the longest history in technology. Glass was being made in Egypt in 1500 BC. Excellent examples of decorative glass have been found that date back to the Roman Empire approximately 2000 years ago. Arrowheads from the Stone Age are obsidian glass found naturally. The basic chemistry of silicate glass formation is as follows. Although, as mentioned above, fused silica can be quenched to a glass, the Tg and Tm of this material are so high (1200°C and 2000°C, respectively) that it is difficult to fabricate. However, the melting point can be lowered considerably by cleavage of some of the silicon–oxygen bonds by heating silica (usually sand) with sodium or potassium oxide or carbonate and calcium oxides. In practice, metal carbonates may be used since they are easier to handle on a large scale and decompose to the oxides at elevated temperatures. The calcium is employed to reduce the solubility of the glass in water. This process lowers the Tg to about 550°C if the material is cooled rapidly, or to 170°C after slow cooling. The melt-processing temperature for good fluidity falls to about 1000°C. The product is window glass or bottle glass, also known as “soda glass” or “soda–lime glass.” This material is inexpensive and chemically and photolytically stable. “Float glass” and fiberglas are essentially the same material. The drawbacks of common soda–lime glass are its brittleness and its relatively low refractive index. It may also be discolored by traces of transition metal impurities (especially iron) which are present in the original sand. Hence, the “white sands” found in various locations around the globe are especially prized for glassmaking.
Note that the nature of the cation used in the formation of glass has an important influence on properties. Monovalent cations such as Na+ or K+ do not form ionic crosslinks between rings or chains. However, Ca2+ or Al3+ are capable of coordination to two or three other chains to form ionic crosslinks. Hence, the multivalent cations may stiffen and strengthen a glass. Transition metal cations such as iron, cobalt, chromium, nickel, or uranium are incorporated into the glass to generate specific colors. This technique has been used for centuries in stained-glass windows and decorative glassware. These glasses are often applied as “glazes” (usually in the form of an emulsion of colored glass particles in water) that are applied to clay objects such as table china or decorative vases, and then heated above the Tg of the glaze to form a coherent film on the surface. Glasses that contain the colored ions uniformly distributed throughout the bulk material are used in optical filters.
The addition of lead or barium oxide or carbonate or other heavy-metal compounds to glass lowers the Tg and increases the refractive index. These high-refractive-index glasses are used in “crystal” drinking glasses, prisms, and lenses. Special low-dispersion glasses used in camera lenses utilize cations such as lanthanide ions.
Note that, although most glasses are chemically inert, cations can be exchanged between the surface regions of the solid and aqueous media. For example, exchange of alkali metal cations may occur, especially if a high surface area of the glass is exposed to the aqueous medium. Lead can be extracted by hot coffee or tea from the glazes used on some ceramic cups and mugs, and this is a well-known health hazard. Soda glass in the form of fiberglass actually dissolves slowly in water because of the high surface area. This was the reason for the now infamous claims for “poly-water” made in the 1960s, which were eventually traced to silicates extracted by water from glass capilliary tubes.
d. Pyrex-Type Glass.
Pyrex glasses are usually based on borosilicates or alumi-nosilicates rather than on traditional silica-derived materials. They are produced by the addition of boric oxide (B2O3) or alumina (Al2O3) to a molten silicate glass at 1600°C. This raises the glass transition temperature and provides resistance to impact and thermal shock. Hence these glasses are used in laboratory glassware and ovenware.
e. Phosphate Glasses.
Phosphoric oxide (P2O5) forms a glass when melted and then cooled. Phosphate glasses have less utility than do silicate glasses. The ultimate crosslinked network consists of PO4 tetrahedra, but with only three of the four oxygens serving as connection points to the surrounding lattice. The fourth oxygen is a nonbonding PO unit. Cleavage of P–O–P bonds by water or aqueous base will reduce the crosslinking until linear polymers or small molecule fragments such as phosphoric acid [O
P(OH)3] are formed. However, the preferred technique to produce phosphate glasses is to dehydrate sodium or potassium phosphate. For example, dehydration of HO–P(O)(ONa)–OH gives linear polymers that form glasses. Phosphate glasses are unstable to water, but fusion with B2O3 or Al2O3 increases the resistance to aqueous media.
f. Borate Glasses.
Borate glasses are of interest because they can be designed to have low thermal expansion coefficients and have relatively low softening temperatures. Hence, they are used as “solder” glasses to join other glasses together in devices such as television cathode ray tubes, and to seal ceramic or metal parts to glass. Boric oxide (B2O3) itself forms a glass after being melted, but many other glasses are formed by the fusion of B2O3 with ZnO, PbO, Tl2O, and La2O2, and these have specialized applications.
g. Fabrication of Glasses.
Most glasses are fabricated by melt techniques. In other words, the material is heated above the melting temperature, or at least above the glass transition temperature, and is then formed into the required shape. Fiber-glas is produced by extrusion of the molten material through fine spinerettes. Float glass with high-quality surfaces is obtained by poring molten glass onto a bath of molten tin (the Pilkinton process). The sheet of glass cools as it moves from one part of the bath to the other and is drawn off in a continous ribbon. Most flat glass is now made by the Pilkinton process. An earlier process (sheet glass) involved drawing a ribbon of solidifying glass from a vat of the molten material. Plate glass is made by extrusion and roller-forming of a sheet of semimolten material, which must then be polished to remove surface imperfections. These processes all require the expenditure of large amounts of energy and massive equipment. By contrast, the sol–gel process described in the next section, which involves the formation of inorganic glass from small-molecule inorganic or organometallic precursors, takes place at lower temperatures and combines synthesis and fabrication.
C. OXIDE CERAMICS FROM SMALL-MOLECULE INORGANIC AND ORGANOMETALLIC PRECURSORS
By contrast to the materials discussed in the last section, we consider here an approach to the preparation of glasses and ceramics that does not involve the direct utilization of mineralogical materials. Instead, for various reasons that will be apparent from the following sections, the properties of the final glass or ceramic can be developed only by starting from simple chemical compounds. Of course, ultimately these are derived from the same mineralogical sources as traditional ceramics, but for reasons of purity or processing control, it is necessary to first convert minerals into pure small-molecule intermediates before synthesis of the ceramic. These additional steps inevitably increase the cost of the final products, but for high-performance applications the additional expense is acceptable.
1. Optical Waveguides (Optical Fibers)
Long-distance telecommunication is now conducted almost entirely over optical fiber networks rather than over the earlier copper wire technology (see Chapter 14). The glass needed as a starting point for the preparation of optical fibers is ultrapure silica. The presence of even minor amounts of colored impurities would reduce the optical path length along the fiber to an unacceptable degree. Hence, mineralogical silica is unsuitable for direct use, and ultra-high-purity silica, produced by chemical synthesis, is needed. This process, which involves the oxidation of highly purified silicon tetrachloride, is described in Chapter 14.
2. The Sol–Gel Process for Low-Temperature Ceramic Formation
The traditional melt fabrication of silicates into shaped glass objects requires the expenditure of large amounts of energy. Moreover, the conventional synthesis of glass uses starting materials obtained with minimal processing from geologic sources, which raises the possibility of contamination by impurities. For these and other reasons an alternative route to amorphous silicates (and other oxide ceramics) has been devised, a process that allows low-temperature processing from pure starting materials.
The usual starting material for this process is a silicon alkoxide such as tetraethy-lorthosilicate, Si(OEt)4 (TEOS), which itself is obtained via the reaction of SiCl4 with ethanol or sodium ethoxide, or by the interaction of sodium ethoxide with silica. The sol–gel process is a controlled hydrolysis of tetraethylsilicate to silica in aqueous alcohol under acidic or basic conditions at or near room temperature. The alkoxide is employed instead of the more easily hydrolyzed SiCl4 because the chloride is converted to silica too rapidly and uncontrollably even in the vapor state. Other alkoxides, such as those of aluminum or titanium, may also be used, and mixtures of two or more alkoxides yield ceramics with a more uniform composition than can be obtained by sintering powders of the oxides. The incorporation of alkoxides of transition elements allows colored glasses to be formed.
A simplified outline of the basic chemistry is shown in reactions 1–4, where R is an ethyl or higher alkyl unit. Any or all of the Si—OR bonds may be hydrolyzed to Si-OH functional groups, and these condense to form Si—O—Si linkages. The reactions can be exceedingly complex and may proceed through the formation of chains or rings, all of which will crosslink at some stage in the reaction sequence. Some control can be exercised through variations in the concentration of the alkoxide, the amount of water in the solvent, the pH, and the type of alkoxy group. For example, the hydrolysis of tetrapropyl silicate is slower than that of tetraethyl silicate. Thus, the linear polymers formed in reaction 1 may undergo further alkoxide hydrolysis to give the crosslinked species also formed in reaction 2, and these crosslinked polymers can further crosslink to give an ultrastructure similar the one formed in reaction 3.
Step (reaction) 1 could also lead to six-, eight- or higher-membered rings instead of chains, and these rings may become joined together to yield clusters of rings (reaction sequence 4). Although cyclic trimeric siloxane rings are shown for simplicity in reaction 4, the most probable products are cyclic tetramers and higher cyclics. Moreover, reaction 4 could also generate cage structures, and these, too, could become linked to form clusters. Subsequently, the alkoxy groups on the outer boundaries of the ring or cage clusters will hydrolyze and provide covalent connections to other clusters to yield larger clusters, and so on. Ring clusters can, in principle, react with chain clusters or linear polymers to increase the structural complexity (Figure 7.14). Eventually a catastrophic gelation of the system will occur, and what was originally a solution or a colloid will become a solvent-swollen solid in the shape of the reaction vessel or mold. Subsequent heating to drive off water and alcohol will complete the condensation process and leave an amorphous form of silica. Note, however, that the removal of water and alcohol as the synthesis and fabrication proceed results in a shrinkage of a shaped object be it a fiber, a lens, or a film. The formation of transparent objects will require a heating to close to the melting point, a step that will result in further shrinkage.
Changes to the pH alter the types of products that are formed, as shown in Figure 7.15. Acidic media, high concentrations of reagents, and lower temperatures favor the formation of chains or loosely crosslinked chains. Basic media, dilute solutions, and higher temperatures favor the formation of rings, cages, and cluster networks.
Figure 7.14. Two different pathways and product strategies that may be followed in sol–gel reactions, depending on reaction conditions.

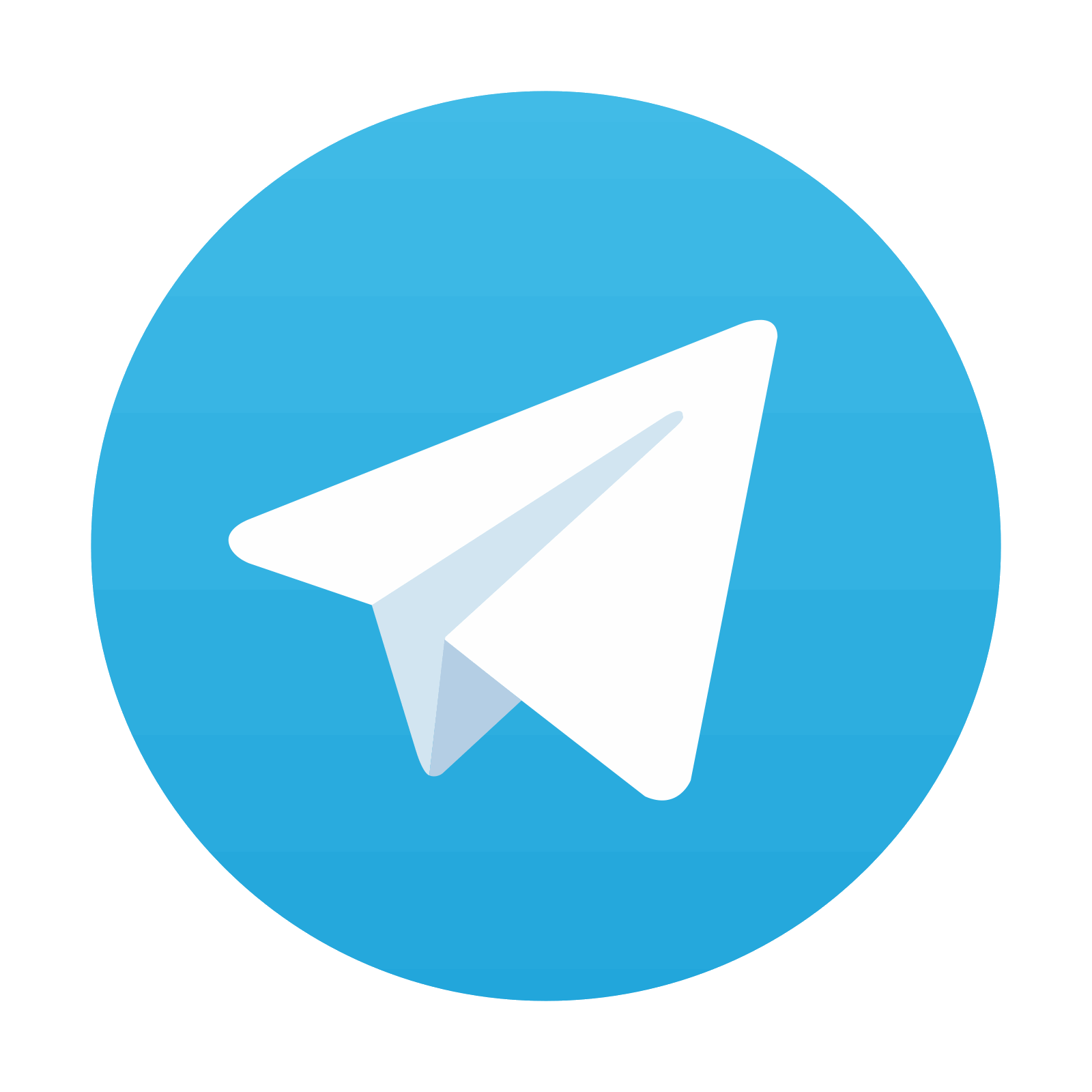
Stay updated, free articles. Join our Telegram channel
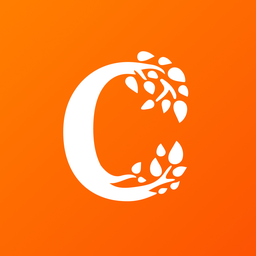
Full access? Get Clinical Tree
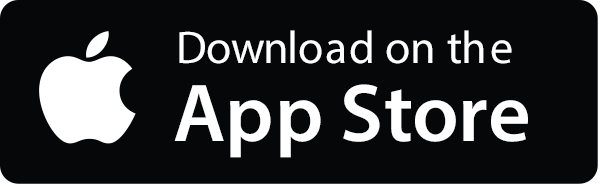
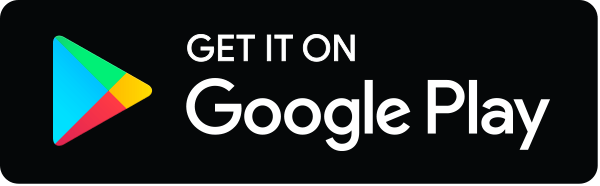