Fig. 20.1
Diagrammatic representation of the imprinting process. Light-colored circles represent undermethylated genes, dark-colored circles represent hypermethylated genes. m maternally derived chromosome, pink. p paternally derived chromosome, blue. See text for details
DNA methylation is involved in human X-chromosome inactivation. Using 5-azacytidine, which causes hypomethylation of DNA, Mohandas et al. were able to achieve reactivation of an inactive human X chromosome [36]. Yen et al. showed that the human HPRT (hypoxanthine phosphoribosyltransferase) gene is hypomethylated on the active X chromosome relative to the inactive X [37]. Furthermore, DNA methylation has been shown, in experiments involving gene insertion into mouse L cells, to render these sequences insensitive to both DNase I and restriction endonucleases, by directing DNA into an inactive supranucleosome structure [38]. These observations suggest that DNA methylation may exert its effect on gene transcription by altering interactions between DNA and nuclear proteins.
The involvement of methylation in the initiation and/or maintenance of genomic imprinting has been examined extensively. Experiments with transgenic mice, in which a foreign gene was inserted into the mouse genome by microinjection, have demonstrated that some transgenes show different states of methylation specific to the parent of origin and that the methylation pattern of those transgenes changes from generation to generation depending upon the sex of the transmitting parent [39–41]. In most cases, a paternally inherited transgene is less methylated than one that is maternally inherited. In a study of transgene-bearing elements of the Rous sarcoma virus (RSV) and a fused c-myc gene, the paternally inherited transgene was undermethylated in all tissues and was expressed only in the heart [41]. This observation suggests that methylation status alone does not determine the expression of a transgene and that undermethylation may be necessary, but not sufficient, for gene expression. In this same study, the somatic organs of a male animal with a maternally inherited transgene exhibited a methylated transgene pattern, but in the testes the transgene was undermethylated, suggesting that the maternally derived methylation pattern is eliminated in the testes of male offspring during gametogenesis.
The role of DNA methylation in genomic imprinting is further demonstrated by observations made in three imprinted endogenous genes in mice: insulin-like growth factor 2 (Igf2), H19 (these two genes are closely linked on mouse chromosome 7) and the Igf2 receptor gene (Igf2r, on mouse chromosome 17).
Studies of mouse H19 showed that it is subject to transcriptional regulation by genomic imprinting, with the maternal allele expressed and the paternal allele silent [42]. By comparing CpG methylation and nuclease sensitivity of chromatin in mouse embryos, Ferguson-Smith et al. showed that hypermethylation and chromatin compaction in the region of the H19 promoter are associated with repression of the paternally inherited copy of the gene [43]. This normally silent paternal H19 allele is activated in DNA methyltransferase-deficient embryos, providing in vivo evidence that a direct correlation is present between DNA methylation and gene activity [44].
Studies of the mouse Igf2 gene showed that, contrary to H19, the paternal allele is expressed in embryos, while the maternal allele is silent, but both parental alleles are transcriptionally active in the choroid plexus and leptomeninges [45]. Therefore, imprinting of Igf2 may also be tissue specific. In addition, studies using mouse embryos with maternal duplication and paternal deficiency of the region of chromosome 7, which encompasses Igf2, showed that the chromatin of the 5′ region of the repressed maternal Igf2 allele is potentially active for transcription; i.e., it is hypomethylated and contains DNase I hypersensitive sites [46]. Recently, a region of paternal-specific methylation between H19 and Igf2 has been postulated to function as the imprint control region. This imprint control region, when unmethylated, acts as a chromatin boundary or insulator that blocks the interaction of Igf2 with its enhancer, thus resulting in silencing of the Igf2 gene, as is observed on the maternal chromosome. On the paternal chromosome, this region is methylated, resulting in the loss of enhancer-blocking activity and allowing the expression of Igf2 [47, 48]. A deletion within this imprint control region results in loss of imprinting of both H19 and Igf2.
Studies of the mouse Igf2r gene indicated that the maternal allele is expressed and the paternal allele is silent [49]. The parental-origin-specific difference in methylation for this gene has been demonstrated in two distinct CpG islands [50]. Here, while the promoter is methylated on the inactive paternal allele, an intronic CpG island is methylated only on the expressed maternal allele, suggesting that methylation of the latter site is necessary for expression of the Igf2r gene.
In humans, the methylation patterns of the parental alleles have been determined for several imprinted loci on chromosome 15 at bands 15q11.2-q13. These include the MKRN3 gene (D15S9) studied in PWS and AS patients and in complete hydatidiform moles, the small nuclear ribonucleoprotein polypeptide N (SNRPN) gene, and the DNA sequence PW71 (D15S63) [51–55]. Distinct differences in methylation of the parental alleles are observed in all instances. This is also true for some of the other known imprinted genes in humans: H19 (maternal allele active) and IGF2 (paternal allele active), both located on the short arm of chromosome 11 at band 11p15.5 [56–58]. In the case of IGF2, although it is the paternal allele that is active, the maternal allele is hypomethylated while the paternal allele is methylated at the 5′ portion of exon 9, similar to the findings in mouse studies. Unlike this gene in mice, the human IGF2R gene is not imprinted [59].
The differentially methylated domains (DMDs) of imprinted genes contain CpG-rich imperfect tandem repeats with similar predicted secondary structures. It is this repeat-related DNA structure, not the sequence, that is implicated in the imprinting mechanism—the establishment and maintenance of parent of origin-specific methylation patterns. It is suggested that a structural feature or features of these tandem repeats are the conserved DMD imprinting signal (reviewed in [35]).
In summary, epigenetic modifications by methylation of alleles of each imprinted gene are established during oögenesis and spermatogenesis. This imprint pattern is maintained throughout embryogenesis and in adult somatic tissues. In fetal gonads, global demethylation of the progenitor germ cells occurs and the inherited imprinting pattern is erased. This is followed by reestablishment of methylation of imprinted genes during gametogenesis, depending on the sex of the fetus. This reprogramming in germ cells ensures that sex-specific genomic imprinting is initiated and that an accurate imprinting cycle is achieved through each generation (reviewed in [60]) (refer to Fig. 20.1).
A difference in DNA replication timing of maternal and paternal alleles of imprinted genes has also been observed [61–65]. Cell-cycle replication timing has been shown to correlate with gene activity: genes that are expressed generally replicate earlier [66, 67]. Furthermore, most genes on homologous chromosomes replicate synchronously [68]. This is not the case for imprinted genes. Using fluorescence in situ hybridization (FISH; see Chap. 17) on interphase nuclei and scoring for the stage of the two alleles in S phase, Kitsberg et al. showed that the imprinted genes H19, Igf2, Igf2r, and Snrpn in mice and their corresponding positions in the human genome all replicate asynchronously, with the paternal allele replicating early [61]. Studies of genes in the 15q11.2-q13 region in humans demonstrated that most show a paternal-early/maternal-late pattern, with some exhibiting the opposite pattern [62, 63]. Therefore, it appears that imprinted genes are embedded in DNA domains with differential replication patterns, which may provide a structural imprint for parental identity [62]. This asynchronous replication timing is established in the gametes during late gametogenesis and maintained throughout development [65].
Thus, the process of genomic imprinting is very complex, and while DNA methylation plays a critical role in genomic imprinting, the process is much more complex than simply inactivating a gene by methylation. It may involve an interaction between DNA methylation, histone modification including acetylation and methylation, chromatin compaction, DNA replication timing, and potentially other mechanisms [69, 70].
Genomic Imprinting and Human Diseases
Genomic imprinting provides an explanation for the observation that the transmission of certain genetic diseases cannot be explained by traditional Mendelian inheritance, but that rather the phenotype depends upon whether the gene involved is maternally or paternally inherited. Conversely, the existence of such diseases provides evidence that genomic imprinting occurs in man. Human conditions that fall into this category include certain deletion/duplication syndromes, a number of cancers, and many disorders arising from uniparental disomy. In addition, imprinted genes may also contribute to modification of disease phenotype, such as is observed in Albright hereditary osteodystrophy, language development, and some psychiatric disorders and complex behavioral phenotypes, including bipolar affective disorder and catatonic schizophrenia [71–74].
Albright hereditary osteodystrophy (AHO) is characterized by short stature, obesity, brachydactyly, mild-to-moderate mental handicap, and subcutaneous ossifications. A proportion of patients with AHO have associated end-organ resistance to parathyroid hormone (PTH), known as “pseudohypoparathyroidism type I” (PHP Ia, also known as AHO with multiple hormone resistance, OMIM #103580). Individuals with AHO and normal endocrine responsiveness have “pseudo-pseudohypoparathyroidism” (PPHP, also known as AHO without multiple hormone resistance, OMIM #612463). AHO is caused by heterozygous deactivating mutation in the GNAS gene located at 20q13. GNAS is imprinted (paternal allele inactive); the imprinting appears to be tissue specific, with maternal expression only in certain cells, such as cells of the proximal renal tubule [75]. In families with AHO, the strongest predictor of the endocrine phenotype is the parent of origin. PHP1A occurs only after maternal inheritance of the molecular defect, either GNAS mutation or GNAS imprinting defects, whereas PPHP occurs only after paternal inheritance of the molecular defect [76]. These observations indicate involvement of imprinting in disease phenotype.
Chromosome Deletion/Duplication Syndromes
Prader-Willi Syndrome/Angelman Syndrome
The best-studied examples of genomic imprinting in human disease are the Prader-Willi and Angelman syndromes. These are clinically distinct disorders; both map to the chromosome 15q11.2-q13 region, but they involve different genes [77–81]. The etiologies of these disorders include the absence of a parent-specific contribution of this region due to either deletion or uniparental disomy (UPD), disruptions in the imprinting process, and mutations within the gene [82–96].
The clinical phenotype of PWS has been well characterized [97, 98]. Briefly, it includes hypotonia during infancy, obesity, hyperphagia, hypogonadism, characteristic facies, small hands and feet, hypopigmentation, and mental deficiency. Approximately 70–75% of cases have an interstitial deletion of a 4 Mb sequence at 15q11.2-q13 on the paternally derived chromosome 15 [69]. Approximately 20–25% of cases are due to maternal uniparental disomy for chromosome 15 and 1% or so as a result of an abnormality of the imprinting process, causing a maternal methylation imprint on the paternal chromosome 15 [86, 89, 93, 94] (Table 20.1). Many paternally expressed transcripts have been identified in a cluster in the proximal part of the 15q11.2-q13 region. These include MKRN3, MAGEL2, NDN, PWRN1, NPAP1, SNURF-SNRPN, a number of C/D box small nucleolar RNA (snoRNA) genes, and other additional transcripts (reviewed in references [99–103]). This clustering of paternally expressed transcripts suggests strong regional control of the imprinting process [103]. It has been recently demonstrated that deficiency of SNORD116 (previously HBII-85) snoRNAs causes the key characteristics of the PWS phenotype [104, 105]. Other imprinted genes in the 15q11.2-q13 region, such as MAGEL2 and NDN, probably also contribute to the PWS phenotype.
Table 20.1
Etiology and recurrence risk of Prader-Willi syndrome and Angelman syndrome
Etiology | PWS | AS | Recurrence risk |
---|---|---|---|
Deletion | ∼70–75% Paternal chr 15 | ∼70% Maternal chr 15 | <1% |
UPD | ∼20–25% Maternal UPD | ∼6% Paternal UPD | <1% |
IC abnormality | ∼1% | ∼3–6% | 50% |
(PWS: when present in father) | |||
(AS: when present in mother) | |||
Gene mutation | – | ∼10% UBE3A | 50% |
(AS: when present in mother) | |||
Unknown | – | ∼10% | – |
The clinical phenotype of AS patients is distinct from that of PWS [106, 107]. Briefly, it includes microcephaly, ataxia, characteristic gait, spontaneous laughter, seizures, severe mental retardation, and hypopigmentation. Approximately 70% of AS patients have a deletion of the same 4 Mb sequence at 15q11.2-q13 on the maternally derived chromosome 15 [83–85]. Approximately 6% are due to paternal uniparental disomy for chromosome 15; 3–6% as a result of an abnormality of the imprinting process, causing a paternal methylation imprint on the maternal chromosome 15; and approximately 10% as a result of a mutation within the AS gene (reviewed in Refs. [81, 87–93, 95, 96, 108, 109]) (Table 20.1). In contrast to PWS, mutation of a single gene, the gene for E6-associated protein (E6-AP) ubiquitin-protein ligase (UBE3A) (maternal allele active) has been identified in some AS families and is considered the candidate gene for AS [81, 96]. The imprinting of UBE3A is tissue specific, being restricted to the brain [110–112]. More recently, another imprinted gene ATP10A, mapped within 250 kb telomeric to UBE3A, has also been shown to be expressed only on the maternal allele [113]. It is speculated that ATP10A may be involved in phospholipid transport and may also contribute to the AS phenotype. Both UBE3A and ATP10A are located at the distal part of the 15q11.2-q13 region.
In both PWS and AS patients with abnormalities of the imprinting process, Buiting et al. identified inherited microdeletions in the 15q11.2-q13 region [114]. They proposed that these deletions probably affect a single genetic element that they called an “imprinting center (IC).” This AS/PWS-IC has been shown to have a bipartite structure and overlaps the SNRPN promoter with the AS-IC being only 35–40 kb upstream of the PWS-IC [115–117]. Mutations or disruptions of the imprinting center impair the imprinting process. These mutations can be transmitted silently through the germline of one parent, the one in whom the gene is normally silent, but appear to block the resetting of the imprint in the germline of the opposite sex. Thus, a female with a PWS-IC mutation will not have affected children. Her sons, however, if they inherit the mutation and are therefore unable to reactivate the cluster of PWS genes in their germ cells, will be at risk of having PWS children, both male and female. The opposite is true for AS; i.e., a male with an AS-IC mutation will not have affected children, but his daughters, if they inherit the mutation, will be at risk of having AS children. These observations in PWS and AS indicate that the PWS genes are active only on the paternal chromosome 15 and the AS gene is active only on the maternal chromosome 15. These two syndromes serve as classical examples of genomic imprinting in humans.
Deletion, UPD, or IC disruption can all result in an abnormal methylation pattern of the PWS/AS parental alleles. Therefore, the most cost-effective approach to laboratory diagnosis of PWS/AS is to perform DNA methylation studies first. This will detect virtually all cases of PWS and approximately 80% of the cases of AS. If the result is abnormal, fluorescence in situ hybridization (FISH) to detect 15q11.2-q13 microdeletion, followed by UPD studies, should be performed to determine the exact etiology. In the case of AS, UBE3A mutation analysis can be considered when the methylation study is normal (Fig. 20.2).


Fig. 20.2
Laboratory diagnostic approaches for Prader-Willi syndrome/Angelman syndrome
Beckwith-Wiedemann Syndrome
Beckwith-Wiedemann syndrome (BWS) is an overgrowth disorder associated with neonatal hypoglycemia, abdominal wall defects, macroglossia, visceromegaly, gigantism, mid-face hypoplasia, and a predisposition to embryonal tumors (seen in 7.5–10% of patients) including Wilms tumor (most common, see next section), rhabdomyosarcoma, and hepatoblastoma [118, 119] (see next section). Most cases (85%) are sporadic. BWS is a multigenic disorder resulting from dysregulation of a number of imprinted genes at the chromosome 11p15.5 region and is caused by several molecular mechanisms. These include:
Cytogenetic abnormalities involving 11p15, present in a small number (∼1%) of all BWS patients. These include duplication of the paternal 11p15 region as a result of either a de novo rearrangement or a familial translocation/inversion and maternally inherited balanced rearrangements involving 11p15 [122–124].
IC mutation in the gene cluster IGF2/H19 or KCNQ1/KCNQ1OT1 [125] (see later). In familial cases, the segregation appears to be autosomal dominant with incomplete penetrance [119]. Furthermore, penetrance appears to be more complete with maternal inheritance; i.e., there is an excess of transmitting females [126, 127].
Linkage studies confirm that BWS maps to 11p15.5 [130, 131]. Imprinted genes in this region have been shown to consist of two domains separated by non-imprinted genes [132, 133]. The proximal centromeric domain contains CDKN1C (p57 KIP2 ) (maternal allele active), KCNQ1 (maternal allele active), and KCNQ1OT1 (LIT1 or KvDMR1) (paternal allele active) [125, 134–136]. The distal telomeric domain contains H19 (maternal allele active) and IGF2 (paternal allele active, located approximately 130 kb centromeric to H19). The paternally expressed genes are growth promoter genes, while the maternally expressed genes are growth suppressor genes. Functional imbalance between the growth promoter and growth suppressor genes causes the phenotype seen in BWS. In some BWS patients who inherited an 11p15.5 allele from both parents, an altered pattern of allelic methylation of H19 and IGF2 has been reported [121, 137]. In these patients, a paternal imprint pattern is seen on the maternal allele, which results in the non-expression of H19, while IGF2 is expressed from both parental alleles. This switching from normally monoallelic expression to biallelic expression is known as loss of imprinting (LOI) and is caused by imprinting center abnormalities. As in PWS/AS, an IC abnormality prevents the resetting of imprinting in the maternal germline and explains the observation that the affected individuals are usually born to carrier mothers in familial cases. The same explanation can be applied to the observation that in BWS patients with balanced rearrangements involving 11p15, the rearrangements are usually maternally inherited. A disruption/mutation of the IC has occurred in the rearrangement process, preventing the resetting of imprinting in the maternal germline, and H19/CDKN1C/KCNQ1 remains inactive on the maternal allele.
In addition to these abnormalities involving 11 p15, other not yet well-defined mechanisms or genetic loci may also cause the BWS phenotype.
Laboratory diagnostic approaches for BWS include cytogenetic analysis to rule out an 11p15 abnormality, UPD study for the 11p15 region, mutation analysis of the CDKN1C gene, and methylation studies of H19/IGF2 and KCNQ1OT1. One study reported that by analyzing the methylation status of the H19 and KCNQ1OT genes in leukocytes, more than 70% of the 97 patients could be diagnosed [138]. Of all cases with abnormal methylation, 80% involved the promoter region of the KCNQ1OT gene and 20% the H19 gene.
Imprinting Disorders and Assisted Reproductive Technology
Assisted reproductive technology (ART), including in vitro fertilization (IVF) and intracytoplasmic sperm injection (ICSI), accounts for 1–3% of all births in developed countries (see Chap. 11). A possible link between ICSI and Angelman syndrome was first reported in 2002 [139]. Two unrelated children conceived by ICSI developed AS. In normal individuals, the maternal SNRPN allele is methylated, and the paternal allele is unmethylated. In these two patients, hypomethylation of the SNRPN promoter region was detected; only an unmethylated band was present by methylation-specific PCR studies, and the normally methylated maternal band was absent. Microdeletion, uniparental disomy, and AS-IC mutation were all excluded as an etiology. The authors suggested that hypomethylation of the SNRPN locus in these two patients resulted from a sporadic imprinting defect on the maternal chromosome. Subsequently, three additional ART-conceived cases of AS were reported: two by ICSI and one using ovarian hyperstimulation alone [140, 141]. These findings are suggestive but not conclusive for an association between ART and AS due to an imprinting defect.
An association between ART and another imprinting disorder, Beckwith-Wiedemann syndrome (see previous section), was reported in 2003. Three case series from BWS registries reported an estimated sixfold increase of BWS cases among children born after ART (reviewed in [142, 143]). More than 60 such BWS patients have been reported. In the vast majority of these BWS patients, loss of methylation at the KCNQ1OT1 gene at 11p15 is observed. Since KCNQ1OT1 is normally maternally methylated/paternally unmethylated, this finding indicates that hypomethylation of the allele on the maternal chromosome is the cause of BWS in these patients. BWS remains the imprinting disorder with the strongest evidence for an association with ART.
A novel imprinting syndrome resulting from maternal hypomethylation at multiple loci was proposed in 2006 [144]. In a cohort of 12 patients with transient neonatal diabetes resulting from loss of maternal methylation at the transient neonatal diabetes locus, the authors found that six patients had hypomethylation at other loci. None of the patients were conceived following ART, although one was born following a period of subfertility. Similarly, studies of BWS patients with loss of maternal methylation at KCNQ1OT1 showed that approximately 25% had hypomethylation at multiple, additional maternally imprinted loci [145, 146]. Again, only a proportion of patients were conceived following ART. These observations suggest that ART is not specifically associated with maternal hypomethylation syndromes.
An association between ART and other imprinting disorders such as Silver-Russell syndrome and retinoblastoma has also been suggested but not established.
The association of imprinting disorders and ART appears to be related to subfertility, ovulation induction, and/or embryo culture. The exact mechanism for the association is not clear. In vitro embryo culture might predispose to loss of methylation. Alternatively, imprinting defects and subfertility might have a common cause, and treatment for infertility with ovarian hyperstimulation may further increase the risk of imprinting defects [141].
To date, the imprinting disorder that has the strongest evidence for an association with ART is BWS. Nevertheless, the absolute risk of BWS after ART is estimated to be <1%; thus, routine screening of BWS in children born after ART does not appear to be warranted [142].
Cancer
Normal epigenetic modifications of DNA involve three types of changes: chromatin modifications, DNA methylation, and genomic imprinting. These are altered in cancer cells. The epigenetic dysregulation in cancer cells includes global genome hypomethylation, regional hypo- and hypermethylation, histone modification, and disturbed genomic imprinting [147, 148]. Thus, an altered genomic imprinting process is a common mechanism for cancer development.
Paraganglioma
A type of non-childhood tumor, paraganglioma (PGL) of the head and neck (glomus tumor), has been mapped to chromosome 11 at two distinct loci, 11q23 and 11q13.1, by linkage analysis [149, 150]. Approximately 30% of cases are familial. Mutation in SDHD (succinate dehydrogenase subunit D), a gene mapped to 11q23 that encodes a mitochondrial respiratory chain protein, has recently been reported in families with PGL [151–153]. Inheritance of PGL is autosomal dominant with both males and females affected. However, transmission is almost exclusively through the father, and only male gene carriers will have affected offspring. The disease is only very rarely observed in the offspring of affected females [149, 154–157]. These observations suggest genomic imprinting. However, expression of SDHD is biallelic (i.e., it is expressed from both maternal and paternal alleles) in all tissues studied to date (lymphoblastoid cell lines, adult brain, fetal brain, and kidney) [151]. Therefore, the mechanism for the observed genomic imprinting inheritance pattern of this tumor is as yet uncertain. It remains possible that imprinting of SDHD is tissue specific and may be restricted to the carotid body, the most common tumor site of PGL, and other paraganglionic cells.
Wilms Tumor/Rhabdomyosarcoma
In a number of embryonal tumors, loss of heterozygosity (LOH) of a specific parental allele has been observed. In all cases studied, the maternal allele is preferentially lost. This suggests that duplication of some paternal alleles results in enhanced cell proliferation, while duplication of certain maternal alleles may inhibit cell proliferation.
In Wilms tumor and rhabdomyosarcoma, LOH involves chromosome 11 [158–160]. LOH does not involve markers for 11p13, the proposed Wilms tumor locus, but only markers on 11p15.5 [159]. Known imprinted genes in the 11p15.5 region include H19, IGF2, and CDKN1C (p57 KIP2 ) (see previous). The expression of CDKN1C is reduced in Wilms tumor [135]. In addition, by using several overlapping subchromosomal transferable fragments from 11p15 distinct from H19 and IGF2, Koi et al. were able to obtain in vitro growth arrest of rhabdomyosarcoma cells [161]. These observations suggest that CDKN1C, which is normally active on the maternal allele only, may be a candidate for a tumor suppressor gene. Loss of the active CDKN1C allele on the maternal chromosome results in tumor development. Besides LOH, another possible mechanism, loss of imprinting (LOI; see BWS), has been proposed. Ogawa et al. reported biallelic IGF2 RNA synthesis in 4 of 30 Wilms tumors they studied [162]. Thus, “relaxation” of IGF2 gene imprinting on the maternal allele has occurred, resulting in its expression. This would be equivalent to having two copies of an active IGF2 gene, as would occur with a paternal duplication or with paternal UPD. A similar biallelic expression of IGF2 was reported in 30% of breast cancer patients studied [163]. Disruption of the imprinting mechanism (i.e., LOI) may therefore also play a role in tumorigenesis. A third possible mechanism has also been proposed in a proportion of Wilms tumor patients. In some patients, LOI was observed in both the Wilms tumor tissue and the normal adjacent kidney tissue, but IGF2 expression was significantly higher in tumor tissue. The overexpression in tumor tissue was accompanied by activation of all four IGF2 promoters [164]. These studies indicate that while genomic imprinting plays an important role in tumorigenesis, a single mechanism does not account for all cases.
Retinoblastoma/Osteosarcoma
In retinoblastoma and osteosarcoma, loss of both functional copies of the retinoblastoma gene (RB1) on chromosome 13 at band q14, usually by mutation or deletion, has been observed [165]. In familial cases, a mutation in one of the alleles is present in the germline. De novo mutations in the germline occur preferentially in the paternal chromosome, consistent with the general observation that new germline mutations arise predominantly during spermatogenesis [166, 167]. In sporadic, nonfamilial tumors, loss of function of both alleles occurs somatically. In sporadic osteosarcomas, the initial mutation occurs preferentially on the paternal chromosome 13, suggesting that genomic imprinting may be involved [168]. In sporadic retinoblastoma, epigenetic change with hypermethylation of the RB1 gene was reported in 9% of cases [169]. RB1 is a tumor suppressor gene, and it has been recently shown to be imprinted [170]. These observations suggest a role of genomic imprinting in retinoblastoma.
Neuroblastoma
Neuroblastoma is the most common extracranial tumor of childhood. Deletions of chromosome 1p and amplification of the MYCN gene on chromosome 2p are frequently seen in neuroblastoma [171]. Preferential amplification of the paternal MYCN allele in neuroblastoma tumor tissues has been reported [172]. In tumors with MYCN amplification, loss of parental 1p alleles was found to be random [172, 173]. In tumors without MYCN amplification, loss of 1p was previously reported to be preferentially maternal (16 of 17 cases) but random in a later study that suggested no imprinted gene in this region [173, 174]. On the other hand, the TP73 tumor suppressor gene located at 1p36.32 has been shown to be imprinted (maternal active) [32].
An imprinted gene cluster at 14q32.2 that includes two closely linked but reciprocally imprinted genes, DLK1 (paternal active) and MEG3 (also known as GTL2) (maternal active), has been identified [175]. These two genes have similarities to IGF2 and H19, genes involved in BWS (see previous section), respectively. Both MEG3 and H19 (gross suppressors) are maternally expressed, and both DLK1 and IGF2 (growth promoters) are paternally expressed. Hypermethylation of the MEG3 promoter differentially methylated region was associated with MEG3 transcriptional repression and was detected in 5 of 20 (25%) neuroblastomas tumors [176]. Therefore, loss of MEG3 expression may also contribute to tumorigenesis in a subset of human cancers.
Uniparental Disomy
The term uniparental disomy (UPD) was introduced by Engel in 1980 [177]. It describes a phenomenon in which both homologs or homologous segments of a chromosome pair are derived from a single parent. An example of the latter is the paternal UPD for 11p15 in BWS described previously. Discussion here will be restricted to uniparental disomies for entire chromosomes, of which there are two types. Uniparental isodisomy describes a state in which both copies of a chromosome are not only derived from one parent but also represent the same homolog (i.e., two copies of the same exact chromosome). Uniparental heterodisomy refers to both of one parent’s homologs being represented (i.e., both chromosomes of the pair from the same parent). The type of UPD present is not always readily apparent, and it should be noted that, because of the recombination that takes place during meiosis, UPD along the length of an involved chromosome pair can be iso- for certain loci and hetero- for others.
UPD for an entire chromosome can occur as a result of gamete complementation, as suggested by Engel [177]. Since aneuploidy is relatively frequent in gametes, the chance union of two gametes, one hypo-, the other hyper-haploid for the same chromosome, will result in a diploid zygote with UPD for that chromosome. Structural rearrangements, such as Robertsonian or reciprocal translocations (see Chap. 9), increase the chance of meiotic malsegregation and thus may predispose to UPD. This is best illustrated by the case reported by Wang et al., in which UPD for chromosome 14 was observed in a child with a paternal (13;14) Robertsonian translocation and a maternal (1;14) reciprocal translocation [177] (see Fig. 20.3 and Chaps. 3 and 9). Studies in animals also support this concept. Maternal or paternal disomies are readily produced in mice with intercrosses between either Robertsonian or reciprocal translocation carriers [14].


Fig. 20.3
An example of paternal UPD formation by gamete complementation. Malsegregation involving chromosome 14 occurred in both parents as the result of structural rearrangements. Mother: reciprocal translocation t(1;14)(q32;q32). Father: Robertsonian translocation der(13;14)(q10;q10). The patient inherited both chromosomes 14 from the father and neither from the mother. Segregation is normal for chromosome 13 in the mother and for chromosome 1 in the father. Chromosomes are Q-banded
Another mechanism for the occurrence of UPD is by “trisomy rescue” [178]. The vast majority of trisomic conceptuses are nonviable; they may survive to term only if one of the trisomic chromosomes is postzygotically lost. In one-third of these cases, such loss will result in UPD in the now disomic cells (Fig. 20.4). Since the loss occurs postzygotically, mosaicism in such conceptuses is often observed, with the trisomic cell line sometimes confined to the placenta (see Chap. 12). Another way of “rescuing” a trisomic conceptus is by forming a smaller marker chromosome from one of the trisomic chromosomes after losing most of its active genetic material. If the one chromosome that rearranged and became the marker chromosome is the single chromosome contributed by one parent, the remaining two of the trisomic chromosomes will be from the same parent and thus represent UPD for this chromosome pair.


Fig. 20.4
A diagrammatic representation of maternal UPD formation by “trisomy rescue.” A trisomic zygote resulting from maternal meiosis I nondisjunction is depicted here. Loss of one of the trisomic chromosomes through either mitotic nondisjunction or anaphase lag results in euploidy. Uniparental disomy occurs in one-third of these cases. m 1 and m 2 maternally derived chromosomes, p paternally derived chromosome
A third possible mechanism for the occurrence of UPD is “monosomy rescue,” the duplication of the single chromosome in monosomic conceptuses [179]. In this case, uniparental isodisomy for the entire chromosome would be observed.
Two mechanisms contribute to the phenotypic effects of UPD. Unmasking of a recessive gene can occur as a result of uniparental isodisomy, in which the disomic chromosomes are homozygous. This was illustrated initially in an individual with cystic fibrosis who had maternal uniparental isodisomy for chromosome 7 and later in many other patients with recessive disorders and UPD (see later in chapter) [179]. The second mechanism is the effect caused by imprinted genes on the involved chromosome. This is best illustrated by PWS/AS patients who have no deletion of 15q11.2, but rather have UPD, as discussed previously. In addition to these two mechanisms, in cases where UPD arises as a result of “trisomy rescue,” the presence of a mosaic trisomic cell line in the placenta and/or fetus may modify the phenotype.
Of the 47 possible types of UPD of whole chromosomes, 36 have been reported to date. Some provide clear evidence for imprinting and some seem to suggest no such effect, while others will require accumulation of additional data before their status in this regard can be determined.
upd(1)mat
At least seven cases of maternal UPD for chromosome 1 have been reported. One patient had lethal autosomal recessive Herlitz-type junctional epidermolysis bullosa as a result of homozygosity for a nonsense mutation in the LAMB3 gene on chromosome 1 [180]. The mother was a heterozygous carrier for the mutation and the father had two normal LAMB3 alleles. The patient died at 2 months of age. Autopsy was not performed but weight and length were reportedly normal, and no overt dysmorphisms or malformations were noted. Another child with Chediak-Higashi syndrome (CHS) was found to be homozygous for a nonsense mutation in the LYST gene for CHS on chromosome 1 [181]. The mother was a carrier of the mutation, while the father had two normal LYST alleles. Two additional unrelated patients had lethal trifunctional protein deficiency due to homozygous alpha-subunit mutations. In both patients, the mothers were heterozygous for the mutation and the fathers did not have the mutation [182]. One patient had Zellweger syndrome due to homozygosity for a maternally inherited mutation in PEX10, one of the peroxisome biogenesis genes [183]. A boy had autism whose mother and brother also had autistic features; the brother did not have UPD [184]. Another case involved a patient with insulin-dependent diabetes mellitus. Maternal UPD for chromosome 1 was accidentally discovered during a family linkage study [185]. This patient was developmentally and mentally normal at age 23. Therefore, maternal UPD for chromosome 1 does not appear to have an imprinting effect.
upd(1)pat
At least seven cases of paternal UPD for chromosome 1 have been reported. A 7-year-old boy presented with pycnodysostosis as a result of a homozygous mutation of the cathepsin K gene, for which the father was a heterozygote and the mother was normal [186]. The child was otherwise developmentally normal. Five additional patients, one with congenital insensitivity to pain with anhidrosis, two with Herlitz junctional epidermolysis bullosa, one with Leber congenital amaurosis, and one with hemolytic-uremic syndrome, were reported [187–191]. All five had paternal isodisomy for chromosome 1. None had any overt dysmorphisms or malformations. Their phenotype resulted from having two copies of the mutated recessive genes, both inherited from their fathers. Another patient was a 43-year-old female with short stature, ptosis, micro-/retrognathia, scoliosis, hearing loss, myopathy, and infertility. She has isochromosomes for the short arm and long arm of chromosome 1 [i(1)(p10),i(1)(q10); see Chaps. 3 and 9] [192]. It was not clear whether the abnormal phenotype in this woman resulted from an imprinting effect or from homozygosity for some undetected recessive alleles. These observations provide no clear evidence for an imprinting effect of paternal UPD 1.
upd(2)mat
Maternal UPD for chromosome 2 has been reported in at least ten cases. Four cases were associated with confined placental mosaicism (CPM) for trisomy 2. Two cases, one with and one without phenotypic abnormalities, resulted from de novo isochromosome formation of the short arm and long arm of chromosome 2 [i(2)(p10),i(2)(q10); see Chaps. 3 and 9]. One case with no phenotypic abnormalities was discovered at age 3 during paternity testing. Three cases had an autosomal recessive disorder due to a maternally inherited homozygous mutation; two of these were unrelated patients with lethal mitochondrial trifunctional protein deficiency as a result of mutations in the HADHA gene, and one patient had infantile-onset ascending spastic paralysis caused by mutations in the gene ALS2 [182, 193–200]. No phenotypic abnormalities were reported other than those associated with the specific autosomal recessive disorders. A common phenotype was observed in the four cases associated with CPM and one of the two cases with isochromosomes. This includes intrauterine growth restriction (IUGR), oligohydramnios, pulmonary hypoplasia, hypospadias (in two patients), and normal development in the four surviving patients at ages 6, 20, 31 months, and 8 years, respectively. IUGR, oligohydramnios, and pulmonary hypoplasia can be explained by placental dysfunction as a result of trisomy 2 mosaicism. However, these same features were also present in one of the two cases with isochromosomes, suggesting a possible imprinting effect of maternal UPD 2 [198]. In another case reported recently, UPD for maternal 2q and paternal 2p was detected in a 36-year-old woman with normal physical and mental development [201]. Therefore, it is not clear whether maternal UPD 2 confers an imprinting effect.
upd(2)pat
At least three cases of paternal isodisomy for the entire chromosome 2 have been reported. A 34-year-old woman diagnosed with retinitis pigmentosa was found to have a homozygous MERTK mutation [190]. She was otherwise phenotypically normal. The patient’s father was heterozygous for the mutation and the mother did not carry the mutation. Two other cases, one with Crigler-Najjar syndrome type I due to a paternally inherited homozygous UGT1A1 mutation at 2q37 and one with Donnai-Barrow syndrome (faciooculoacousticorenal syndrome) due to homozygous mutation in the LRP2 gene at chromosome 2q31.1, had no phenotypic abnormalities other than the disease-related findings [202, 203]. These cases further illustrate unmasking of autosomal recessive disorders as a result of uniparental disomy. An additional case with isodisomy for paternal 2p as described under upd(2)mat was phenotypically normal. Paternal UPD 2 therefore does not appear to have an imprinting effect.
upd(3)mat
At least two cases of maternal isodisomy for chromosome 3 have been reported. Both cases presented with autosomal recessive disorder as a result of homozygous mutations: one dystrophic epidermolysis bullosa with no additional phenotypic abnormalities and one Fanconi-Bickel syndrome, a rare disorder with growth failure, hepatomegaly, renal Fanconi syndrome, and abnormal glucose homeostasis caused by mutations in SLC2A2 (previously GLUT2) at 3q26.1-3q26.3 [204, 205]. Another case with confined placental mosaicism (CPM, see Chap. 12) for trisomy 3 detected by chorionic villous biopsy and a marker of chromosome 3 origin detected by a subsequent amniocentesis was found to have maternal uniparental disomy for the two chromosomes 3 [206]. The fetus had IUGR and microcephaly that could be attributed to CPM and the marker chromosome. There is no clear evidence that maternal UPD 3 has an imprinting effect.
upd(3)pat
A case of paternal UPD for chromosome 3 was detected serendipitously during a whole genome linkage study [207]. No apparent phenotypic abnormality was observed.
upd(4)mat
A case of maternal UPD for chromosome 4 as a result of isochromosome formation of the short arm and long arm of chromosome 4 [i(4)(p10),i(4)(q10); see Chaps. 3 and 9] was reported in an abstract [208]. Cytogenetic studies were performed because of multiple early miscarriages. The patient was otherwise phenotypically normal. Two additional cases of maternal isodisomy have been reported: one patient had afibrinogenemia as a result of a maternally inherited homozygous mutation of the fibrinogen alpha-chain gene at 4q28; the other adult female patient had a history of major depressive disorder and multiple suicide attempts but normal fertility and no major medical complaints [209, 210]. Another case with confined placental mosaicism for trisomy 4 in a fetus with intrauterine growth restriction and oligohydramnios followed by intrauterine fetal death at 30 weeks of gestation was determined to have maternal UPD 4 [211]. No external malformations were detected in this stillborn. There is no clear evidence to date that maternal UPD for chromosome 4 confers an imprinting effect.
upd(5)pat
Paternal UPD for chromosome 5 was reported in a child with autosomal recessive spinal muscular atrophy [212]. The child had no other developmental abnormalities. Spinal muscular atrophy in this case can be explained by the paternal transmission of two copies of the defective gene. There is no evidence for an imprinting effect.
upd(6)mat
Maternal uniparental isodisomy for chromosome 6 was first identified in a renal transplant patient in the process of HLA typing [213]. Another patient with congenital adrenal hyperplasia resulting from unmasking of the maternally inherited mutation in the 21-hydroxylase gene had intrauterine growth restriction but good catch-up growth [214]. There is no clear evidence for an imprinting effect.
upd(6)pat
More than 15 cases of paternal uniparental disomy for chromosome 6 have been reported (reviewed in references [215–219]). All except one were isodisomy. Many patients had transient neonatal diabetes mellitus (TNDM) associated with very low birth weight. Two genes, an imprinted cell cycle control gene PLAGL1 (also known as ZAC) and hydatidiform mole associated and imprinted gene HYMAI (untranslated with unknown function) at 6q24 with differential methylation of parental alleles, have been identified [220–222]. These genes are expressed only from the paternal allele and are potential candidate genes for TNDM. Increased expression of this gene by paternal UPD appears to result in the diabetic phenotype. It was estimated that paternal UPD 6 accounts for approximately 15–20% of cases of TNDM [221, 223]. Paternal UPD 6 clearly has an imprinting effect.
upd(7)mat
More than 60 patients with maternal UPD for chromosome 7 have been reported in the literature [27, 224–227]. This was the first documented UPD in humans, identified initially in two individuals with cystic fibrosis and short stature [179, 228]. Approximately 10% of patients with Silver-Russell syndrome (SRS) are noted to have maternal UPD 7 [225, 226, 229, 230]. SRS is a heterogeneous disorder. The clinical phenotype includes intrauterine growth restriction and persistent postnatal growth delay, body asymmetry, triangular face, prominent forehead, decreased subcutaneous tissue, delayed bone age, and usually normal intelligence.
Three regions on chromosome 7 have been shown to contain imprinted genes [231, 232]. One region at 7p12 contains an imprinted gene GRB10 (growth factor receptor-binding protein 10), a known growth suppressor that is expressed on the maternal allele and is therefore one of the candidate genes for SRS [233–235]. A second region at 7q32.2 contains a number of other imprinted genes including CPA4, MEST (also known as PEG1), and COPG2IT1. CPA4 was proposed to be a candidate for SRS [236]. The role of the other genes is not yet clear [236–239]. A third region at 7q21.3 contains three imprinted genes SGCE (epsilon-sarcoglycan, maternally imprinted), PEG10, and PPP1R9A. Mutation of the SGCE gene causes an autosomal dominant movement disorder myoclonus-dystonia. A 36-year-old man with both SRS and myoclonus-dystonia has been reported [232].
SRS is also the first human disorder with imprinting disturbances that affect two different chromosomes: chromosomes 7 and 11 [240]. Approximately 40% of SRS show hypomethylation in the IC region upstream of H19 at 11p15.5, which results in overexpression of the growth suppressor H19. Hypermethylation of the same IC region, on the other hand, is associated with the overgrowth disorder, BWS (see section “Chromosome Deletion/Duplication Syndromes”). These two developmental syndromes, SRS and BWS, are therefore clinically and epigenetically opposite diseases (reviewed in [241]).
Maternal UPD 7 clearly has an imprinting effect.
upd(7)pat
At least four cases of paternal isodisomy for the entire chromosome 7 have been reported. One patient had recessive congenital chloride wasting diarrhea with normal growth and development [242]. One patient had cystic fibrosis as a result of inheriting two copies of the ΔF508 mutation from his father. This patient also had complete situs inversus and immotile cilia with growth retardation and significant respiratory disease [243]. The other two patients also had cystic fibrosis. One of them had normal growth and the other had overgrowth and developmental delay [244, 245]. In addition, two patients had paternal isodisomy 7p and maternal isodisomy 7q [246, 247]. These two patients had similar phenotypes that resembled the phenotype seen in maternal UPD 7, and their growth retardation was considered to be a result of maternal isodisomy for 7q. It is not clear whether paternal UPD 7 confers an imprinting effect.
upd(8)mat
One case of maternal isodisomy for the entire chromosome 8 has been reported [248]. The patient was a 39-year-old male with normal appearance, stature, and intelligence. He had early-onset ileal carcinoid, slight thoracic scoliosis, and numerous pigmented nevi. More cases are needed before a conclusion can be drawn as to whether maternal UPD 8 has an imprinting effect.
upd(8)pat
A single case of paternal uniparental isodisomy for chromosome 8 has been reported [249]. This five-and-half-year-old girl had normal development and lipoprotein lipase (LPL) deficiency due to a mutation of the LPL gene. The patient was ascertained due to a diagnosis of chylomicronemia. The father was a heterozygous carrier for the same mutation. It appears that normal development can occur in paternal UPD 8 and that an imprinting effect of this UPD may not exist.
upd(9)mat
At least seven cases of maternal UPD for chromosome 9 have been reported. Two patients had recessive cartilage-hair hypoplasia, a disorder that maps to the short arm of chromosome 9 [250]. Two homozygotic female twins had Leigh syndrome as a result of inheriting two copies of the mutated SURF1 gene from their mother [251]. Both twins died of respiratory failure at age 3. No gross dysmorphic features or malformations were noted apart from Leigh syndrome. One case involved a fetus associated with confined placental mosaicism (see Chap. 12) for trisomy 9 [252]. Pathological examination of the abortus was not possible. One case of syndromic congenital hypothyroidism, characterized by thyroid dysgenesis, cleft palate, spiky hair, and choanal atresia and bifid epiglottis who was homozygous for a maternally inherited FOXE1 (9q22) mutation, has been reported recently [253]. Another 34-year-old healthy woman with recurrent spontaneous abortions had isochromosomes of the short and long arms of chromosome 9 [i(9)(p10),i(9)(q10); see Chaps. 3 and 9]. Molecular analysis demonstrated maternal isodisomy [254]. The available data indicate that maternal UPD 9 may not have an imprinting effect.
upd(10)mat
A case of prenatally diagnosed maternal UPD for chromosome 10 associated with confined placental mosaicism (see Chap. 12) has been reported [255]. The infant was phenotypically and developmentally normal at 8 months of age. Another case of familial hemophagocytic lymphohistiocytosis characterized by lethal primary immunodeficiency was reported in a patient who was homozygous for the perforin gene (PRF1) at 10q22 as a result of maternal uniparental disomy for chromosome 10 [256]. Two other cases of maternal UPD 10 reported were associated with either a marker chromosome 10 or a trisomy 10 cell line, and the abnormal phenotypes were attributed to the karyotypic abnormalities. There is no evidence to date that this UPD confers an imprinting effect.
upd(11)pat
Paternal UPD for the entire chromosome 11 has been reported in at least three cases. One patient had hemihypertrophy, congenital adrenal carcinoma, and Wilms tumor [257]. The second had associated confined placental mosaicism (see Chap. 12) for trisomy 11, and intrauterine death occurred between 19 and 20 weeks gestation. This fetus had growth restriction, aberrant intestinal rotation, and hypospadias [258]. The third patient had possible mosaic paternal isodisomy along the entire chromosome 11. The clinical findings in this patient did not differ from that of other BWS patients [259]. In addition, many cases of paternal segmental UPD for distal 11p associated with BWS have been observed (see previous section). The existence of an imprinting effect due to paternal UPD 11 is clear.
upd(12)mat
A case of maternal UPD for chromosome 12 was reported [260]. The infant had normal somatic and psychomotor development with no congenital anomalies or dysmorphic features at 6 weeks of age. Chromosome analysis demonstrated mosaicism with the presence in some cells of a small marker chromosome consisted of chromosome 12 centromeric heterochromatin with no euchromatic material. This suggests that the mechanism for the occurrence of UPD in this case is by trisomy rescue. It appears that maternal UPD 12 may not have an imprinting effect.
upd(13)mat
upd(13)pat
At list six cases of paternal UPD for chromosome 13 have been reported. One was the mother of one of the maternal UPD 13 patients described previously [261]. This phenotypically normal individual presumably received the isochromosome 13q from her father, who was not available for study, but DNA polymorphism studies of her mother revealed the absence of maternal chromosome 13 alleles in this patient [263]. Four cases had de novo der(13;13)(q10;q10) translocations (see Chaps. 3 and 9) [264–266]. Three of them were complete isodisomies, and one exhibited evidence of recombination with proximal isodisomy and distal heterodisomy. All four patients were phenotypically normal. Another case of maternal UPD 13 was reported in a patient with hearing loss as a result of unmasking of the recessive gap junction protein GJB2 gene at 13q11-q12 [266]. Therefore, paternal UPD 13 does not appear to have an imprinting effect.
upd(14)mat
Maternal UPD for chromosome 14 has been reported in many cases (reviewed in [267–275]). Although many are associated with Robertsonian translocations involving chromosome 14, cases with a normal karyotype have also been observed. A distinct clinical phenotype is present and consists of intrauterine growth restriction, mild-to-moderate motor and/or mental developmental delay, hypotonia, short stature, and precocious puberty. Less frequent findings include hydrocephalus, dysmorphic features (prominent forehead, supraorbital ridge, short philtrum, downturned corner of mouth), small hands, hyperextensible joints, scoliosis, and recurrent otitis media.
Chromosome 14 contains a cluster of imprinted genes at 14q32.2 including RTL1 and DLK1 (both paternal active) and MEG3 (also known as GTL2, maternal active) (see also previous “Neuroblastoma” section). They are regulated by a differentially methylated region (DMR) between RTL1/DLK1 and MEG3 genes (intergenic DMR). A number of patients with biparental inheritance of chromosome 14 but with a clinical phenotype similar to that of maternal uniparental disomy have been found to have various deletions in the imprinted region or hypomethylation of the intergenic and the MEG3 DMRs [273–278]. These findings indicate that abnormal methylation patterns of the imprinted genes are associated with the maternal UPD 14 phenotype. Therefore, methylation analysis of the imprinted gene MEG3 can be performed to detect upd(14)mat and to determine the molecular basis in patients with a phenotype similar to upd(14)mat but who do not have UPD [273, 274].
Evidence for an imprinting effect due to maternal UPD 14 is clear.
upd(14)pat
Many cases of paternal UPD for chromosome 14 have been reported [279–284]. Most are associated with Robertsonian translocations involving chromosome 14; cases with a normal karyotype have also been observed [285]. A similar phenotype is present in these patients and includes polyhydramnios, low birth weight, hirsute forehead, blepharophimosis/short palpebral fissures, protruding philtrum, small ears, small thorax, abnormal ribs, simian creases, and joint contractures. Severe mental retardation was seen in a patient who was beyond 20 months of age at the time of reporting [279]. These observations indicate that an imprinting effect due to paternal UPD 14 exists.
Studies comparing maternal and paternal UPD cases with cases of partial trisomy and partial monosomy of various segments of 14q have suggested that 14q23-q32 may be the region where the imprinted genes on chromosome 14 reside [286, 287]. Further studies of segmental and full paternal isodisomy for chromosome 14 indicated imprinted genes at 14q32 as the critical components of the phenotype observed in upd(14)pat [288]. This is consistent with the observation that overexpression of the paternally active gene RTL1 plays a major role in the upd(14)pat phenotype [277] (see also previous section discussing maternal UPD 14).
Human chromosome 14 has significant homology to mouse chromosomes 12 and 14 [289]. Mouse chromosome 12 is imprinted, and both maternal and paternal disomies cause early embryonic death [290]. Thus, the observation of imprinting effects for both maternal and paternal UPD 14 in humans is not unexpected.
upd(15)mat
More than 100 cases of maternal UPD for chromosome 15 have been reported in the literature in association with Prader-Willi syndrome [86, 89, 291, 292] (see section “Prader-Willi Syndrome/Angelman Syndrome in Genomic Imprinting and Human Diseases”). As previously discussed, UPD(15)mat accounts for approximately 20–25% of patients with PWS. Many patients had associated trisomy 15 mosaicism, which was confined to the placenta in most cases. Comparison of the phenotypes of PWS patients with different etiologies has shown that advanced maternal age was present in mothers of patients with maternal UPD, while a higher frequency of hypopigmentation is seen in patients due to deletion of paternal 15q11.2-q13 [291–293]. Advanced maternal age can be expected in UPDs that result from “trisomy rescue,” as advanced maternal age is associated with meiotic nondisjunction. Hypopigmentation results from mutation/deletion of the OCA2 gene (mouse homolog pink-eyed dilution p gene) located at 15q11.2-q12 [294–296]. The human OCA2 gene is not imprinted, and both copies are functional in UPD patients. Hypopigmentation is therefore more prominent in PWS patients due to deletion. Differences in other clinical features between these two groups are less clear-cut. While there may not be a significant difference in the overall severity, female UPD patients were found to be less severely affected than female deletion patients [291]. UPD patients were found to be less likely to have “typical” facial appearance, somewhat higher IQ, and milder behavior problems and more likely to have psychosis and autism spectrum disorders [292, 297, 298]. Deletion patients have a higher frequency of sleep disturbance, hypopigmentation, and speech articulation defects [299]. These differences can again be attributed, at least partially, to the presence of two copies of non-imprinted genes in UPD cases, whereas there is haploinsufficiency of these genes in the deletion cases.
upd(15)pat
Many cases of paternal UPD for chromosome 15 associated with Angelman syndrome have been reported in the literature [88, 90, 300–302]. Paternal UPD 15 accounts for approximately 6% of AS patients. AS patients with paternal UPD may have a milder phenotype than those with a maternal deletion of 15q11.2-q13; UPD patients have better physical growth, fewer movement abnormalities, less ataxia, and a lower prevalence of seizures [90, 300–303]. One possible mechanism for the milder phenotype in UPD patients may be the presence of many non-imprinted genes in the 15q11.2-q13 region in these patients, whereas these are absent in deletion patients. Alternatively, as proposed by Bottani et al., it may be due to the “leaky” expression of the imprinted paternal genes, where two copies of the allele will result in an expression higher than in deletion cases, in which only one imprinted paternal allele is present [90].
Both maternal and paternal UPD 15 clearly confer imprinting effects.
upd(16)mat
More than 20 cases of maternal UPD for chromosome 16 have been described, and potentially many more cases are not reported [304–312]. Again, associated trisomy 16 mosaicism, usually confined to the placenta, is present in most cases. A clinical phenotype of maternal UPD 16 has not been clearly defined; the possibility of the presence of an undetected trisomy 16 cell line complicates the comparison among reported cases. IUGR is a frequent finding. IUGR may result from the presence of trisomy 16 cells in the placenta; however, no catch-up growth was observed in these patients [304]. Development has been normal in all cases, the oldest reported at 4 years of age. Imperforate anus has been reported in three cases, hypospadias in two, and congenital cardiac anomalies were observed in five cases, with an A-V canal defect in one and ASD and VSD in four. Subtle but apparently characteristic facial dysmorphisms (slightly upslanted palpebral fissures, almond-shaped eyes, broad nasal root, upturned nares, long philtrum, thin upper lip, prominent ears, and triangular face) may exist [307, 309, 311]. In addition, in a later study, statistical analysis performed on a large series of mosaic trisomy 16 cases with molecular determination of UPD status indicated that upd(16)mat was associated with fetal growth restriction and with increased risk of major malformation [313]. One protein coding gene, ZNF597 at 16p13.3, has been found to be imprinted (maternal allele active) [32]. Although not yet certain, the existence of an imprinting effect due to maternal UPD 16 is a distinct possibility.
upd(16)pat
A single case of paternal UPD for chromosome 16 has been reported [314]. This case was associated with confined placental mosaicism (see Chap. 12). Paternal isodisomy for chromosome 16 was prenatally diagnosed and confirmed after birth. Intrauterine growth restriction was present with catch-up growth observed at 13 months of age. Minor physical abnormalities included bilateral pes calcaneus and additional rudimentary mandibular dental arch. Psychomotor development was normal. It is not clear whether paternal UPD 16 has an imprinting effect.
upd(17)mat
A case of maternal UPD involving the entire chromosome 17 was reported in a 2-year-old boy with trisomy 17 confined placental mosaicism (see Chap. 12) [315]. His growth and psychomotor development was normal. Another case with infantile nephropathic cystinosis as a result of a homozygous 57-kb deletion encompassing the CTNS gene at 17p13 was recently reported [316]. The mother was heterozygous for the deletion and the father did not carry the deletion. The child had maternal UPD for chromosome 17, and the abnormal phenotype was resulted from unmasking of the recessive gene. There is no evidence that maternal UPD 17 confers an imprinting effect.
upd(20)mat
At least three cases of maternal UPD 20 have been reported [317–319]. One of them was associated with a mosaic cell line containing a small marker chromosome consisting of the pericentromeric region of chromosome 20, and another was associated with confined placental mosaicism (see Chap. 12) for trisomy 20. The common features in these three patients at ages 4 years, 35, and 17 months, respectively, are pre- and postnatal growth retardation. Isolated findings included mild facial dysmorphism, strabismus, microcephaly, macrocephaly, developmental delay, and hyperactivity. A further case involved a live-born girl with maternal uniparental isodisomy of chromosome 20 in the diploid cell line who had moderate psychomotor retardation, central hypotonia with peripheral hypertonia, multiple minor dysmorphism, and marked kyphosis [320]. However, the clinical phenotype in this patient was complicated by the presence of a trisomy 20 cell line in both blood and urine specimens. Imprinted genes have been found on chromosome 20; available clinical information in the literature unfortunately does not unequivocally support the possibility that maternal UPD 20 has an imprinting effect [32].
upd(20)pat
No pure paternal UPD involving the entire chromosome 20 has been reported. One case had a structurally abnormal chromosome 20 derived from a terminal rearrangement that joined two chromosomes 20 at band p13 [45,XY,psu dic(20;20)(p13;p13)] [321]. DNA polymorphism studies indicated that the two chromosomes 20 in this terminal rearrangement were derived from one paternal chromosome, thereby representing paternal isodisomy. The patient had multiple anomalies including microtia/anotia, micrencephaly, congenital heart disease, neuronal subependymal heterotopias, and colonic agangliosis. However, this case was complicated by the presence of trisomy 20 cells in skin. Therefore, although an imprinting effect is possible for paternal UPD 20, a definitive conclusion cannot be drawn without further case reports.
upd(21)mat
Maternal UPD for chromosome 21 has been reported in at least two patients [322, 323]. Both had a balanced de novo (21;21) Robertsonian translocation (see Chaps. 3 and 9) and were phenotypically normal. Although maternal UPD 21 has been reported in early abortus specimens, it has not been possible to clearly attribute embryonal death to UPD [324]. Therefore, maternal UPD 21 may be considered at this time to have no imprinting effect.
upd(21)pat
upd(22)mat
Maternal UPD for chromosome 22 not associated with mosaic trisomy 22 has been reported in three cases [327–329]. All three phenotypically normal individuals were ascertained via history of multiple spontaneous abortions and were found to have balanced (22;22) Robertsonian translocations (see Chaps. 3 and 9). Two other cases of maternal uniparental heterodisomy for chromosome 22 associated with prenatally diagnosed mosaic marker chromosome 22 were reported: one male infant had no dysmorphic features at birth and reportedly normal development at age 6 months and the other, a girl, had normal development at 18 months of age [330, 331]. Additionally, a prenatally diagnosed case with nonmosaic trisomy 22 in placental tissue and apparently nonmosaic normal 46,XY cells in newborn blood had severe intrauterine growth restriction, first-degree hypospadias, and other features attributed to prematurity [332]. There is no evidence that maternal UPD 22 has an imprinting effect.
upd(22)pat
upd(X)mat
Maternal UPD for the two X chromosomes in females has been reported in three cases [334, 335]. The first two cases were detected by screening a normal population of 117 individuals. The third patient had Duchenne muscular dystrophy due to homozygosity of a maternally inherited deletion of exon 50 of the dystrophin gene. These observations indicate that maternal UPD for the X chromosome may not have an imprinting effect. To date, no imprinted genes on the X chromosome have been identified in humans.
upd(X)pat
A single case of paternal UPD for the two X chromosomes in the 46,XX cell line of a 14-year-old girl with 45,X/46,XX mosaicism (see Chap. 10) has been reported [336]. This patient had impaired gonadal function and short stature. The presence of a 45,X cell line makes it difficult to determine if the observed clinical features in this patient can be attributed to paternal UPD for the X chromosome. Therefore, it is unknown at this time if paternal UPD X has an imprinting effect. However, no imprinted genes on the X chromosome have been identified in humans.
upd(XY)pat
A single case of paternal contribution of both the X and Y chromosomes in a male patient was reported in an abstract [337]. This patient was ascertained because he had hemophilia A, which was transmitted from his father. No abnormalities other than hemophilia were described. Paternal UPD for XY may therefore not have an imprinting effect.
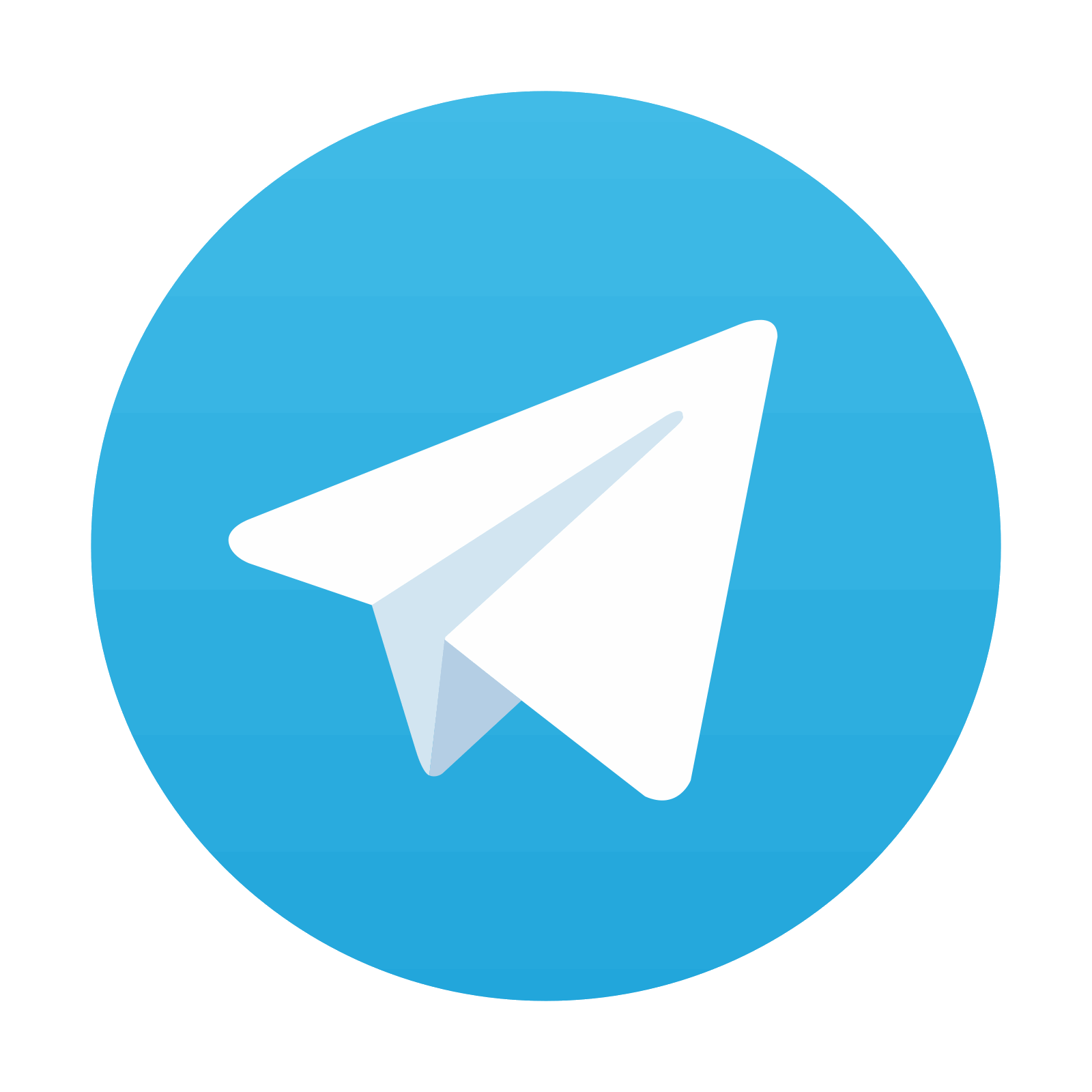
Stay updated, free articles. Join our Telegram channel
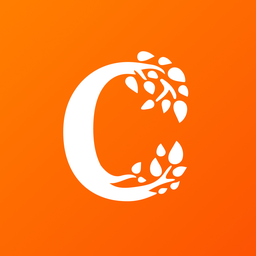
Full access? Get Clinical Tree
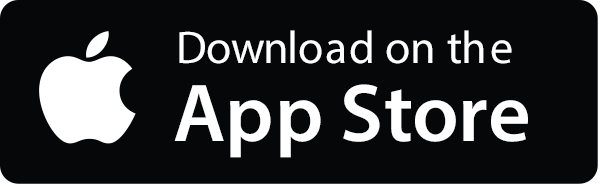
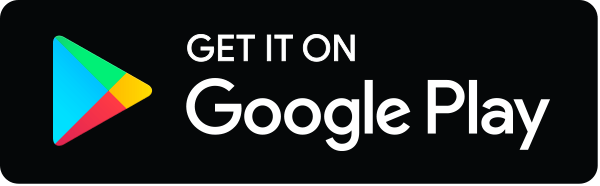