protein, which is necessary for cellular structure or function and contributes to a particular inherited trait, such as eye color or blood type. In the cell, a gene is transcribed within the nucleus into messenger ribonucleic acid (mRNA). This message leaves the nucleus and many different chemicals and cellular components within the cell cytoplasm are used to translate mRNA into an amino acid chain. A specific cellular component, called a ribosome, reads the message of three mRNA bases at a time. A sequence of three mRNA bases is called a codon, and there are 64 different codons within our genetic code. The ribosome reads the mRNA codons to determine and assemble the sequence of amino acids that will undergo further modification within the cell to become a functional protein. (See How genes control cell function, page 96.)
![]() |
condition in which a person reflexively sneezes when quickly transitioning from a dark environment to intense bright light is thought to be dominant. A person who doesn’t experience this has two recessive alleles, whereas a person who does reflexively sneeze has either one or both dominant alleles for the trait.
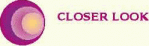
![]() |
in somatic cells during early embryogenesis. This phenomenon is called X-inactivation or Lyonization. X-inactivation ensures that females, like males, have one functional copy of the X chromosome in each body cell. The process of inactivating either the paternally contributed X or the maternally contributed X is random in each cell. Due to X-inactivation, one recessive allele will be expressed in some somatic cells and the partner allele (whether dominant or recessive) in other somatic cells. The most common example occurs not in people but in cats. Only female cats have calico (tricolor) coat patterns. Dark and orange hair color in the cat is carried on the X chromosome. Some hair cells in females express the brown allele and others, the orange color. The white color results from an autosomal gene.
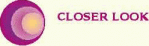
![]() |
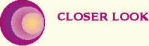
![]() |
![]() |
![]() |
![]() |
![]() |
cases, the father is affected and the mother is a carrier. All daughters of an affected male will be carriers. Sons of an affected male will be unaffected, and the unaffected sons aren’t carriers. Unaffected male children of a female carrier don’t transmit the disorder. Hemophilia is an example of an X-linked inheritance disorder. (See X-linked recessive inheritance.)
![]() |
![]() |
Teratogens | Associated disorders |
Infections | |
Toxoplasmosis Rubella Cytomegalovirus Herpes simplex Other infections (syphilis, hepatitis B, mumps, gonorrhea, parvovirus, varicella) | ♦ Growth deficiency ♦ Mental retardation ♦ Hepatosplenomegaly ♦ Hearing loss ♦ Cardiac and ocular defects ♦ Active infection ♦ Carrier state |
Maternal disorders | |
Diabetes mellitus | ♦ Abnormalities of the spine, lower extremities, heart, kidney, or external genitalia |
Phenylketonuria | ♦ Mental retardation ♦ Microcephaly ♦ Congenital heart defects ♦ Intrauterine growth retardation |
Hyperthermia | ♦ Intrauterine growth retardation ♦ Central nervous system (CNS) and neural tube defects ♦ Facial defects |
Drugs, chemicals, and physical agents | |
Alcohol | ♦ Fetal alcohol syndrome ♦ Learning disabilities |
Anticonvulsants | ♦ Intrauterine growth retardation ♦ Mental deficiency ♦ Facial abnormalities ♦ Cardiac defects ♦ Cleft lip and palate ♦ Malformed ears ♦ Genital defects |
Cocaine | ♦ Premature delivery ♦ Abruptio placentae ♦ Intracranial hemorrhage ♦ GI and genitourinary (GU) abnormalities |
Diethylstilbestrol | ♦ Clear-cell adenocarcinoma of vagina ♦ Structural and functional defects of female GU tract |
Folate deficiency | ♦ Neural tube defects |
Lithium | ♦ Congenital heart disease |
Methotrexate | ♦ Intrauterine growth retardation ♦ Decreased ossification of skull ♦ Prominent eyes ♦ Limb abnormalities ♦ Mild developmental delay |
Radiation | ♦ Microcephaly ♦ Mental retardation |
Tetracycline | ♦ Brown staining of decidual teeth ♦ Dental caries ♦ Enamel hypoplasia |
Vitamin A derivatives | ♦ Facial defects ♦ Cardiac defects ♦ CNS defects ♦ Incomplete development of thymus |
Warfarin (Coumadin) | ♦ Intrauterine growth retardation ♦ Mental retardation ♦ Seizures ♦ Nasal hypoplasia ♦ Abnormal calcification of axial skeleton |
Adapted from Hansen, M. Pathophysiology: Foundations of Disease and Clinical Intervention. Philadelphia: W.B. Saunders, 1998, with permission of the publisher. |
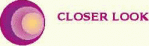
![]() |


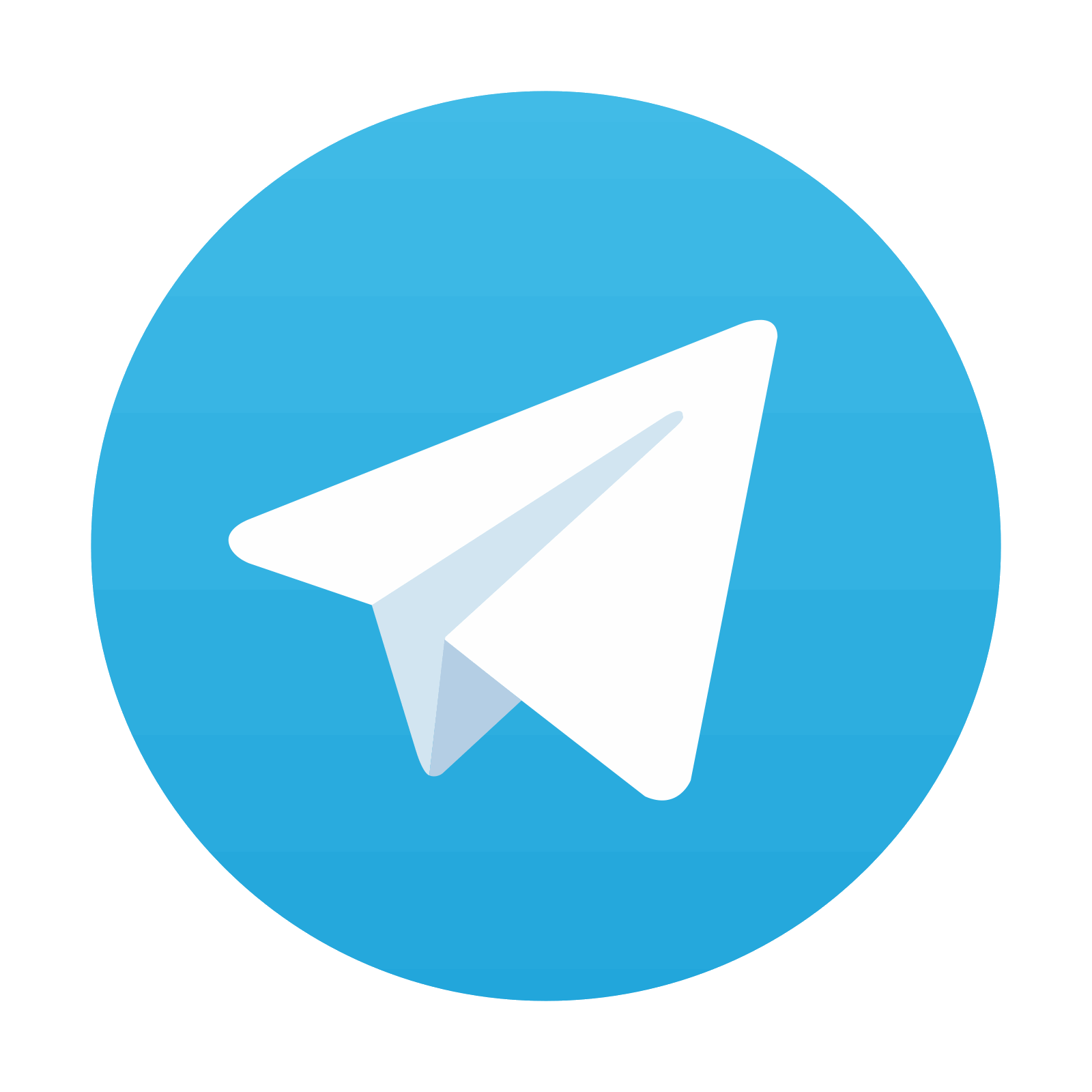
Stay updated, free articles. Join our Telegram channel
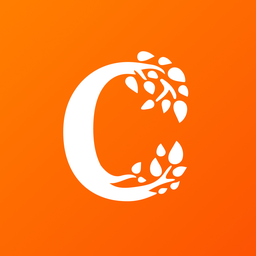
Full access? Get Clinical Tree
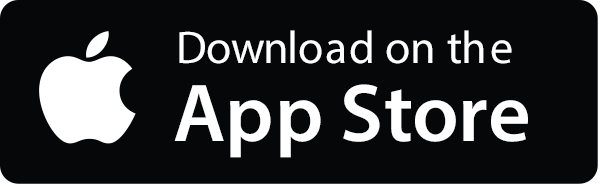
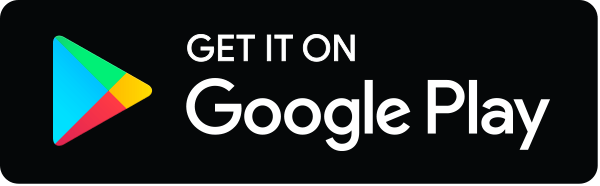
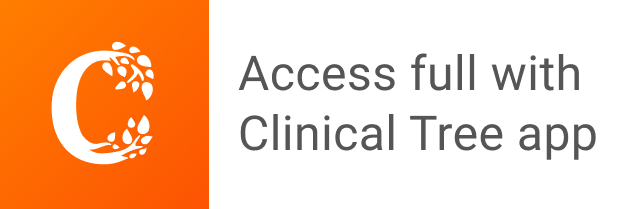