Glucose is the universal fuel for human cells. Every cell type in the human is able to generate adenosine triphosphate (ATP) from glycolysis, the pathway in which glucose is oxidized and cleaved to form pyruvate. The importance of glycolysis in our fuel economy is related to the availability of glucose in the blood, as well as the ability of glycolysis to generate ATP in both the presence and absence of O2. Glucose is the major sugar in our diet and the sugar that circulates in the blood to ensure that all cells have a continuous fuel supply. The brain uses glucose almost exclusively as a fuel.
Glycolysis begins with the phosphorylation of glucose-to-glucose 6-phosphate (glucose 6-P) by hexokinase (HK). In subsequent steps of the pathway, one glucose 6-P molecule is oxidized to two pyruvate molecules with the generation of two molecules of reduced nicotinamide adenine nucleotide (NADH) (Fig. 22.1). A net generation of two molecules of ATP occurs through direct transfer of high-energy phosphate from intermediates of the pathway to adenosine diphosphate (ADP) (substrate-level phosphorylation).
FIGURE 22.1 Overview of glycolysis and the TCA cycle. Acetyl CoA, acetyl coenzyme A; ADP, adenosine diphosphate; ATP, adenosine triphosphate; Fructose 6-P, fructose 6-phosphate; Fructose-1,6-bis P, fructose 1,6-bisphosphate; Glucose 6-P, glucose 6-phosphate; NADH, reduced nicotinamide adenine dinucleotide; Pi, inorganic phosphate; TCA, tricarboxylic acid.
Glycolysis occurs in the cytosol and generates cytosolic NADH. Because NADH cannot cross the inner mitochondrial membrane, its reducing equivalents are transferred to the electron transport chain by either the malate–aspartate shuttle or the glycerol 3-phosphate shuttle (see Fig. 22.1). Pyruvate is then oxidized completely to CO2 by pyruvate dehydrogenase and the tricarboxylic acid (TCA) cycle (see Chapter 23). Complete aerobic oxidation of glucose to CO2 can generate approximately 30 to 32 mol of ATP per mole of glucose.
When cells have a limited supply of oxygen (e.g., the kidney medulla), or few or no mitochondria (e.g., the red cell), or greatly increased demands for ATP (e.g., skeletal muscle during high-intensity exercise), they rely on anaerobic glycolysis for generation of ATP. In anaerobic glycolysis, lactate dehydrogenase oxidizes the NADH generated from glycolysis by reducing pyruvate to lactate (Fig. 22.2). Because O2 is not required to reoxidize the NADH, the pathway is referred to as anaerobic. The energy yield from anaerobic glycolysis (2 mol ATP per mole of glucose) is much lower than the yield from aerobic oxidation. The lactate (lactic acid) is released into the blood. Under pathological conditions that cause hypoxia, tissues may generate enough lactic acid to cause lactic acidemia.
FIGURE 22.2 Anaerobic glycolysis (shown in red). The conversion of glucose to lactate generates 2 ATP from substrate-level phosphorylation. Because there is no net generation of reduced nicotinamide adenine dinucleotide (NADH), there is no need for O2, and thus the pathway is anaerobic. Acetyl CoA, acetyl coenzyme A; ATP, adenosine triphosphate; TCA, tricarboxylic acid.
In each cell, glycolysis is regulated to ensure that ATP homeostasis is maintained, without using more glucose than necessary. In most cell types, HK, the first enzyme of glycolysis, is inhibited by glucose 6-phosphate (see Fig. 22.1). Thus, glucose is not taken up and phosphorylated by a cell unless glucose 6-P enters a metabolic pathway, such as glycolysis or glycogen synthesis. The control of glucose 6-P entry into glycolysis occurs at phosphofructokinase-1 (PFK-1), the rate-limiting enzyme of the pathway. PFK-1 is allosterically inhibited by ATP and allosterically activated by adenosine monophosphate (AMP). AMP increases in the cytosol as ATP is hydrolyzed by energy-requiring reactions.
Glycolysis has functions in addition to ATP production. For example, in liver and adipose tissue, this pathway generates pyruvate as a precursor for fatty acid biosynthesis. Glycolysis also provides precursors for the synthesis of compounds such as amino acids and five-carbon sugar phosphates.
While glucose is at the center of carbohydrate metabolism and is the major dietary sugar, other sugars in the diet are converted to intermediates of glucose metabolism, and their fates parallel that of glucose.
Fructose, the second most common sugar in the adult diet, is ingested principally as the monosaccharide or as part of sucrose (Fig. 22.3). It is metabolized principally in the liver (and to a lesser extent in the small intestine and kidney) by phosphorylation at the 1-position to form fructose 1-phosphate (fructose 1-P), followed by conversion to intermediates of the glycolytic pathway. The major products of its metabolism in liver are, therefore, the same as for glucose (including lactate, blood glucose, and glycogen). Essential fructosuria (fructokinase deficiency) and hereditary fructose intolerance (a deficiency of the fructose 1-P cleavage by aldolase B) are inherited disorders of fructose metabolism.
FIGURE 22.3 Fructose. The sugar fructose is found in the diet as the free sugar in foods such as honey or as a component of the disaccharide sucrose in fruits and sweets. It also can be synthesized from glucose via the polyol pathway. In the lens of the eye, the polyol pathway contributes to the formation of cataracts. Fructose is metabolized by conversion to intermediates of glycolysis.
Fructose synthesis from glucose in the polyol pathway occurs in seminal vesicles and other tissues. Aldose reductase converts glucose to the sugar alcohol sorbitol (a polyol), which is then oxidized to fructose. In the lens of the eye, elevated levels of sorbitol in diabetes mellitus may contribute to the formation of cataracts.
Galactose is ingested principally as lactose, which is converted to galactose and glucose in the intestine. Galactose is converted to glucose principally in the liver. It is phosphorylated to galactose 1-phosphate (galactose 1-P) by galactokinase and activated to a uridine diphosphate (UDP)-sugar by galactose 1-phosphate uridylyltransferase. The metabolic pathway subsequently generates glucose 1-phosphate (glucose 1-P). Classical galactosemia, a deficiency of galactose 1-phosphate uridylyltransferase, results in the accumulation of galactose 1-phosphate in the liver and the inhibition of hepatic glycogen metabolism and other pathways that require UDP-sugars. Cataracts can occur from the accumulation of galactose in the blood, which is converted to galactitol (the sugar alcohol of galactose) in the lens of the eye.
THE WAITING ROOM 
Linda F. is a 68-year-old woman who is admitted to the hospital emergency room with very low blood pressure (80/40 mm Hg) caused by an acute hemorrhage from a previously diagnosed ulcer of the stomach. Her bleeding stomach ulcer has reduced her effective blood volume severely enough to compromise her ability to perfuse (deliver blood to) her tissues. She is also known to have chronic obstructive pulmonary disease (COPD) as a result of 42 years of smoking two packs of cigarettes per day. Her respiratory rate is rapid and labored, her skin is cold and clammy, and her lips are slightly blue (cyanotic). She appears anxious and moderately confused.
As appropriate emergency measures are taken to stabilize her and elevate her blood pressure, blood is sent for immediate blood typing and crossmatching, so that blood transfusions can be started. A battery of laboratory tests is ordered, including venous hemoglobin, hematocrit, and an arterial blood gas, which includes an arterial pH, partial pressures of oxygen (pO2) and carbon dioxide (pCO2), bicarbonate, and oxygen saturation. Results show that the hemorrhaging and COPD have resulted in hypoxemia, with decreased oxygen delivery to her tissues, and both a respiratory and metabolic acidosis.
Otto S., a 26-year-old medical student, had gained weight during his first sedentary year in medical school. During his second year, he began watching his diet, jogging for an hour four times each week, and playing tennis twice a week. He has decided to compete in a 5-km race. To prepare for the race, he begins training with wind sprints, bouts of alternately running and walking.
Ivan A. is a 56-year-old morbidly obese accountant (see Chapters 1 through 3). He decided to see his dentist because he felt excruciating pain in his teeth when he ate ice cream. He really likes sweets and keeps hard candy in his pocket. The dentist noted from Ivan A.’s history that he had numerous cavities as a child in his baby teeth. At this visit, the dentist found cavities in two of Ivan A.’s teeth.
Candice S. is an 18-year-old girl who presented to her physician for a precollege physical examination. While taking her medical history, the doctor learned that she carefully avoided eating all fruits and any foods that contained table sugar. She related that from a very early age, she had learned that these foods caused severe weakness and symptoms suggestive of low blood sugar such as tremulousness and sweating. Her medical history also indicated that her mother had told her that once she started drinking and eating more than breast milk, she became an irritable baby who often cried incessantly, especially after meals, and vomited frequently. At these times, her abdomen had become distended, and she became drowsy and apathetic. Her mother had intuitively eliminated certain foods from Candice S.’s diet, after which the severity and frequency of these symptoms diminished.
Erin G. is the third child in her family, with a normal pregnancy and vaginal delivery at home like her older siblings. Her mother was unable to get to the initial pediatrician visit because she was busy with all her young children but noticed that Erin G. began vomiting 3 days after birth, usually within 30 minutes after breastfeeding and finally brought her to the pediatrician at 3 weeks when she noticed her eyes were yellow. She also reported her abdomen became distended at these times, and she became irritable and cried frequently. The doctor agreed that Erin G. was slightly jaundiced. He also noted an enlargement of her liver and questioned the possibility of early cataract formation in the lenses of Erin G.’s eyes. He ordered liver and kidney function tests and did two separate dipstick urine tests in his office, one designed to measure only glucose in the urine and the other capable of detecting any of the reducing sugars.
I. Glycolysis
Glycolysis is one of the principal pathways for generating ATP in cells and is present in all cell types. The central role of glycolysis in fuel metabolism is related to its ability to generate ATP with, and without, oxygen. The oxidation of glucose to pyruvate generates ATP from substrate-level phosphorylation (the transfer of phosphate from high-energy intermediates of the pathway to ADP) and NADH. Subsequently, the pyruvate may be oxidized to CO2 in the TCA cycle and ATP generated from electron transfer to oxygen in oxidative phosphorylation (see Chapter 23). However, if the pyruvate and NADH from glycolysis are converted to lactate (anaerobic glycolysis), ATP can be generated in the absence of oxygen, via substrate-level phosphorylation.
Glucose is readily available from our diet, internal glycogen stores, and the blood. Carbohydrate provides 50% or more of the calories in most diets, and glucose is the major carbohydrate. Other dietary sugars, such as fructose and galactose, are oxidized by conversion to intermediates of glycolysis. Glucose is stored in cells as glycogen, which can provide an internal source of fuel for glycolysis in emergency situations (e.g., decreased supply of fuels and oxygen during ischemia, due to a reduced blood flow). Insulin and other hormones maintain blood glucose at a constant level (glucose homeostasis), thereby ensuring that glucose is always available to cells that depend on glycolysis for generation of ATP.
After a high-carbohydrate meal, glucose is the major fuel for almost all tissues. Exceptions include intestinal mucosal cells, which transport glucose from the gut into the blood, and cells in the proximal convoluted tubule of the kidney, which return glucose from the renal filtrate to the blood. During fasting, the brain continues to oxidize glucose because it has a limited capacity for the oxidation of fatty acids or other fuels. Cells also continue to use glucose for the portion of their ATP generation that must be met by anaerobic glycolysis, due to either a limited oxygen supply or a limited capacity for oxidative phosphorylation (e.g., the red blood cell).
In addition to serving as an anaerobic and aerobic source of ATP, glycolysis is an anabolic pathway that provides biosynthetic precursors. For example, in liver and adipose tissue, this pathway generates pyruvate as a precursor for fatty acid biosynthesis. Glycolysis also provides precursors for the synthesis of compounds such as amino acids and of nucleotides. The integration of glycolysis with other anabolic pathways is discussed in Chapter 34.
A. The Reactions of Glycolysis
The glycolytic pathway, which cleaves 1 mol of glucose to 2 mol of the three-carbon compound pyruvate, consists of a preparative phase and an ATP-generating phase. In the initial preparative phase of glycolysis, glucose is phosphorylated twice by ATP to form fructose 1,6-bisphosphate (Fig. 22.4). The ATP expenditure in the beginning of the preparative phase is sometimes called “priming the pump,” because this initial utilization of 2 mol of ATP per mole of glucose results in the production of 4 mol of ATP per mole of glucose in the ATP-generating phase.
FIGURE 22.4 Phases of the glycolytic pathway. ATP, adenosine triphosphate; Fructose-1,6-bis P, fructose 1,6-bisphosphate; NADH, reduced nicotinamide adenine dinucleotide.
In the ATP-generating phase, fructose 1,6-bisphosphate is split into two triose phosphates. Glyceraldehyde 3-phosphate (a triose phosphate) is oxidized by NAD+ and phosphorylated using inorganic phosphate. The high-energy phosphate bond generated in this step is transferred to ADP to form ATP. The remaining phosphate is also rearranged to form another high-energy phosphate bond that is transferred to ADP. Because 2 mol of triose phosphate were formed, the yield from the ATP-generating phase is 4 ATP and 2 NADH. The result is a net yield of 2 mol of ATP, 2 mol of NADH, and 2 mol of pyruvate per mole of glucose.
1. Conversion of Glucose-to-Glucose 6-Phosphate
Glucose metabolism begins with the transfer of a phosphate from ATP to glucose to form glucose 6-P (Fig. 22.5). Phosphorylation of glucose commits it to metabolism within the cell because glucose 6-P cannot be transported back across the plasma membrane. The phosphorylation reaction is irreversible under physiological conditions because the reaction has a high negative ∆G0′. Phosphorylation does not, however, commit glucose to glycolysis.
FIGURE 22.5 Glucose 6-P metabolism. ADP, adenosine diphosphate; ATP, adenosine triphosphate; glucose 6-P, glucose 6-phosphate.
Glucose 6-P is a branch point in carbohydrate metabolism. It is a precursor for almost every pathway that uses glucose, including glycolysis, the pentose phosphate pathway (see Chapter 27), and glycogen synthesis (see Chapter 26). From the opposite point of view, it also can be generated from other pathways of carbohydrate metabolism, such as glycogenolysis (breakdown of glycogen), the pentose phosphate pathway, and gluconeogenesis (the synthesis of glucose from noncarbohydrate sources).
Hexokinases, the enzymes that catalyze the phosphorylation of glucose, are a family of tissue-specific isoenzymes that differ in their kinetic properties. The isoenzyme found in liver and β-cells of the pancreas has a much higher Km than other HKs and is called glucokinase. In many cells, some of the HK is bound to porins in the outer mitochondrial membrane (voltage-dependent anion channels; see Chapter 24), which gives these enzymes first access to newly synthesized ATP, as it exits the mitochondria.
2. Conversion of Glucose 6-P to the Triose Phosphates
In the remainder of the preparative phase of glycolysis, glucose 6-P is isomerized to fructose 6-phosphate (fructose-6-P), again phosphorylated, and subsequently cleaved into two three-carbon fragments (Fig. 22.6). The isomerization, which positions a keto group next to carbon 3, is essential for the subsequent cleavage of the bond between carbons 3 and 4.
FIGURE 22.6 Reactions of glycolysis. High-energy phosphates are indicated by the red squiggles. ADP, adenosine diphosphate; ATP, adenosine triphosphate; NAD+, nicotinamide adenine dinucleotide; Pi, inorganic phosphate.
The next step of glycolysis, phosphorylation of fructose-6-P to fructose 1,6-bis-phosphate (fructose 1,6-bis-P) by phosphofructokinase-1 (PFK-1), is generally considered the first committed step of the pathway. This phosphorylation requires ATP and is thermodynamically and kinetically irreversible. Therefore, PFK-1 irrevocably commits glucose to the glycolytic pathway. PFK-1 is a regulated enzyme in cells, and its regulation controls the entry of glucose into glycolysis. Like HK, it exists as tissue-specific isoenzymes whose regulatory properties match variations in the role of glycolysis in different tissues.
Fructose 1,6-bis-P is cleaved into two phosphorylated three-carbon compounds (triose phosphates) by aldolase (see Fig. 22.6). Dihydroxyacetone phosphate (DHAP) and glyceraldehyde 3-phosphate (G3P) are the products. DHAP is isomerized to G3P by triose phosphate isomerase. Aldolase is named for the mechanism of the forward reaction, which is an aldol cleavage, and the mechanism of the reverse reaction, which is an aldol condensation. The enzyme exists as tissue-specific isoenzymes, which all catalyze the cleavage of fructose 1,6-bis-P but differ in their specificities for fructose 1-P. The enzyme uses a lysine residue at the active site to form a covalent bond with the substrate during the course of the reaction. Inability to form this covalent linkage inactivates the enzyme.
Thus, at this point in glycolysis, for every mole of glucose that enters the pathway, 2 mol of glyceraldehyde 3-P are produced and continue through the pathway at the expense of two high-energy bonds.
3. Oxidation and Substrate-Level Phosphorylation
In the next part of the glycolytic pathway, glyceraldehyde 3-P is oxidized and phosphorylated so that subsequent intermediates of glycolysis can donate phosphate to ADP to generate ATP. The first reaction in this sequence, catalyzed by glyceraldehyde 3-P dehydrogenase, is really the key to the pathway (see Fig. 22.6). This enzyme oxidizes the aldehyde group of glyceraldehyde 3-P to an enzyme-bound carboxyl group and transfers the electrons to NAD+ to form NADH. The oxidation step is dependent on a cysteine residue at the active site of the enzyme, which forms a high-energy thioester bond during the course of the reaction. The high-energy intermediate immediately accepts an inorganic phosphate to form the high-energy acyl phosphate bond in 1,3-bisphosphoglycerate, releasing the product from the cysteine residue on the enzyme. This high-energy phosphate bond is the start of substrate-level phosphorylation (the formation of a high-energy phosphate bond where none previously existed, without the utilization of oxygen).
In the next reaction, catalyzed by phosphoglycerate kinase, the phosphate in this high-energy bond is transferred to ADP to form ATP. The energy of the acyl phosphate bond is high enough (~10 kcal/mol) so that transfer to ADP is an energetically favorable process. 3-Phosphoglycerate is also a product of this reaction.
To transfer the remaining low-energy phosphoester on 3-phosphoglycerate to ADP, it must be converted into a high-energy bond. This conversion is accomplished by moving the phosphate to the second carbon (forming 2-phosphoglycerate) and then removing water to form phosphoenolpyruvate (PEP). The enolphosphate bond is a high-energy bond (its hydrolysis releases ~14 kcal/mol of energy), so the transfer of phosphate to ADP by pyruvate kinase is energetically favorable (see Fig. 22.6) and not reversible. This final reaction converts PEP to pyruvate.
4. Summary of the Glycolytic Pathway
The overall net reaction in the glycolytic pathway is
Glucose + 2 NAD+ + 2 Pi + 2 ADP → 2 pyruvate + 2 NADH + 4 H+ + 2 ATP + 2 H2O
The pathway occurs with an overall negative ∆G0′ of approximately −22 kcal/mol. Therefore, it cannot be reversed without the expenditure of energy.
B. Fructose
Fructose is found in the diet as a component of sucrose in fruit, as a free sugar in honey, and in high-fructose corn syrup (see Fig. 22.3). Fructose enters epithelial cells and other types of cells by facilitated diffusion on the GLUT V transporter. It is metabolized to intermediates of glycolysis. Problems with fructose absorption and metabolism are relatively more common than with other sugars.
1. Fructose Metabolism
Fructose is metabolized by conversion to glyceraldehyde-3-P and dihydroxyacetone phosphate, which are intermediates of glycolysis (Fig. 22.7). The steps parallel those of glycolysis. The first step in the metabolism of fructose, as with glucose, is phosphorylation. Fructokinase, the major kinase involved, phosphorylates fructose at the 1-position. Fructokinase has a high Vmax and rapidly phosphorylates fructose as it enters the cell. The fructose 1-phosphate formed is not an intermediate of glycolysis but rather is cleaved by aldolase B to dihydroxyacetone phosphate (an intermediate of glycolysis) and glyceraldehyde. Glyceraldehyde is then phosphorylated to glyceraldehyde-3-phosphate by triose kinase. Dihydroxyacetone phosphate and glyceraldehyde 3-phosphate are intermediates of the glycolytic pathway and can proceed through it to pyruvate, the TCA cycle, and fatty acid synthesis. Alternatively, these intermediates can also be converted to glucose by gluconeogenesis. In other words, the fate of fructose parallels that of glucose.
FIGURE 22.7 Fructose metabolism. The pathway for the conversion of fructose to dihydroxyacetone-P and glyceraldehyde-3-P is shown in red. These two compounds are intermediates of glycolysis and are converted in the liver principally to glucose, glycogen, or fatty acids. In the liver, aldolase B cleaves both fructose-1-P in the pathway for fructose metabolism, and fructose-1,6-BP in the pathway for glycolysis. ADP, adenosine diphosphate; ATP, adenosine triphosphate; dihydroxyacetone-P, dihydroxyacetone phosphate; Fructose-6-P, fructose 6-phosphate; fructose-1,6-BP, fructose 1,6-bisphosphate; Glucose-1-P, glucose 1-phosphate; Glucose-6-P, glucose 6-phosphate; glyceraldehyde-3-P, glyceraldehyde 3-phosphate; TCA, tricarboxylic acid.
The metabolism of fructose occurs principally in the liver and to a lesser extent in the small intestinal mucosa and proximal epithelium of the renal tubule because these tissues have both fructokinase and aldolase B. Aldolase exists as several isoforms: aldolases A, B, C, and fetal aldolase. Although all of these aldolase isoforms can cleave fructose 1,6-bisphosphate, the intermediate of glycolysis, only aldolase B can also cleave fructose 1-phosphate. Aldolase A, present in muscle and most other tissues, and aldolase C, present in brain, have almost no ability to cleave fructose 1-phosphate. Fetal aldolase, present in the liver before birth, is similar to aldolase C.
Aldolase B is the rate-limiting enzyme of fructose metabolism, although it is not a rate-limiting enzyme of glycolysis. It has a much lower affinity for fructose 1-phosphate than fructose 1,6-bisphosphate and is very slow at physiologic levels of fructose 1-phosphate. As a consequence, after ingesting a high dose of fructose, normal individuals accumulate fructose 1-phosphate in the liver while it is slowly converted to glycolytic intermediates. Individuals with hereditary fructose intolerance (a deficiency of aldolase B) accumulate much higher amounts of fructose 1-phosphate in their livers.
Other tissues also have the capacity to metabolize fructose but do so much more slowly. The HK isoforms present in muscle, adipose tissue, and other tissues can convert fructose-to-fructose 6-phosphate but react much more efficiently with glucose. As a result, fructose phosphorylation is very slow in the presence of physiologic levels of intracellular glucose and glucose 6-phosphate.
2. Synthesis of Fructose in the Polyol Pathway
Fructose can be synthesized from glucose in the polyol pathway. The polyol pathway is named for the first step of the pathway, in which sugars are reduced to the sugar alcohol by the enzyme aldose reductase (Fig. 22.8). Glucose is reduced to the sugar alcohol sorbitol, and sorbitol is then oxidized to fructose. This pathway is present in seminal vesicles, which synthesize fructose for the seminal fluid. Spermatozoa use fructose as a major fuel source while in the seminal fluid and then switch to glucose once in the female reproductive tract. Utilization of fructose is thought to prevent acrosomal breakdown of the plasma membrane (and consequent activation), while the spermatozoa are still in the seminal fluid.
FIGURE 22.8 The polyol pathway converts glucose to fructose. NAD+, nicotinamide adenine dinucleotide.
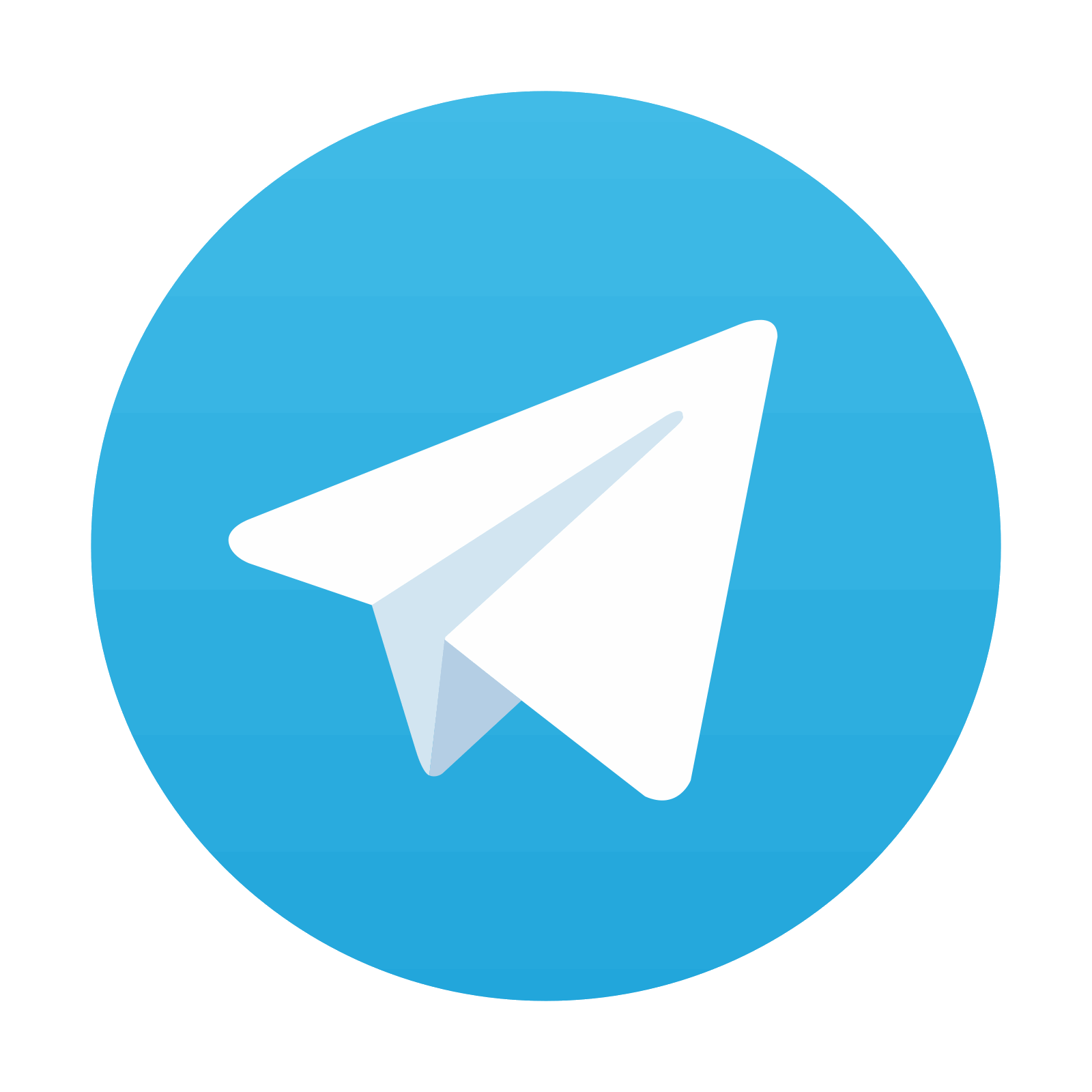
Stay updated, free articles. Join our Telegram channel
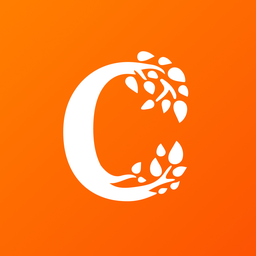
Full access? Get Clinical Tree
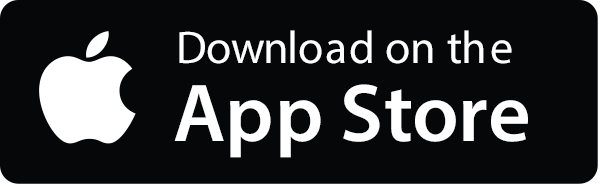
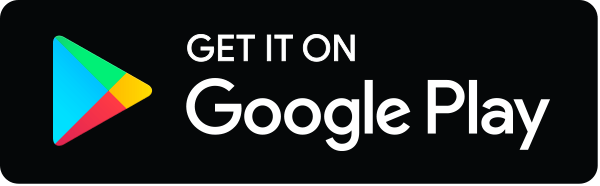