CHAPTER 2 General Characteristics of the Spine
FUNCTION AND DEVELOPMENT OF THE SPINE
The anatomy of the human spine can be understood best if its functions are considered first. The spine has three primary functions: support of the body, protection of the spinal cord and spinal nerve roots, and movement of the trunk. The vertebral column has the ideal structure to carry out all of these functions simultaneously (Putz and Müller-Gerbl, 1996). These varied functions are carried out by a series of movable bones, called vertebrae, and the soft tissues that surround these bones. A brief explanation of the development of the vertebrae and the related soft tissues is given to highlight the detailed anatomy of these structures. A more thorough discussion of spinal development is presented in Chapter 12.
Development of the Spine
After the early development of the neural groove into the neural tube and neural crest (see Fig. 12-7), paraxial mesoderm condenses to form somites (see Figs. 12-7 and 12-9, A). The somites, in turn, develop into dermomyotomes and sclerotomes. Portions of the lateral aspects of the dermomyotomes develop into the dermis and subcutaneous tissue, whereas the majority of the dermomyotomes develop into the axial musculature. The sclerotomes migrate centrally to surround the neural tube and notochord (see Fig. 12-9, B). The sclerotomal cells then form the vertebral column and associated ligaments.
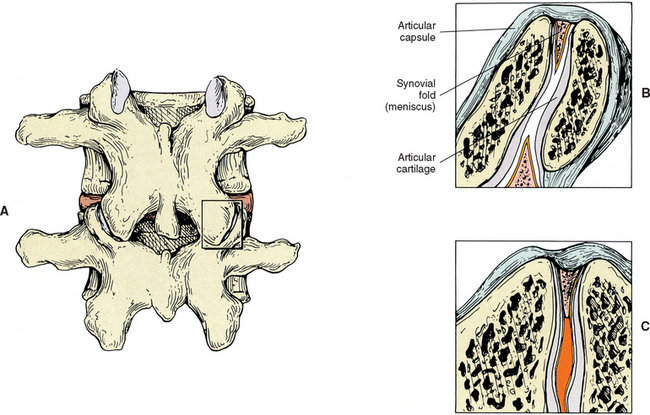
FIG. 2-9 Z joint synovial folds. A, Posterior view of the lumbar Z joint. B, A coronal section deep to that demonstrated in A. This coronal section shows the Z joint synovial folds. Notice the synovial lining of these folds, the articular cartilage, and the joint space. The synovial fold is attached to the articular capsule. C, An entrapped synovial fold. The distal portion of the fold is fibrous, and the proximal portion contains vessels and adipose tissue. Giles and Taylor (1987) also have found sensory nerve endings within the Z joint synovial folds.
Chondrification Centers and Primary Ossification Centers.
Cells of sclerotomal origin condense to form vertebral chondrification centers (one pair in the anterior aspect and at least one center in each half of the posterior aspect of the mesenchymal vertebrae). This results in the development of a cartilage model of each vertebra (see Fig. 12-11). Each vertebra then develops three primary centers of ossification (see Fig. 12-11). One primary center is located in the anterior part of the future vertebra. This region is known as the centrum and helps to form the future vertebral body. The remaining two primary ossification centers are located on each side of the portion of the vertebra that surrounds the developing neural tube. This region is known as the neural or posterior arch. The two ossification centers at the neural arch normally unite posteriorly to form the spinous process. Failure of these centers to unite results in a condition known as spina bifida. This condition is discussed in more detail in Chapter 12.
The precise time of fusion between the neural arch and centrum at the neurocentral synchondrosis remains a topic of current investigation. Some authors state that closure occurs by 6 years of age (Maat et al., 1996), and other investigators state that the neurocentral cartilage may remain until as late as 16 years of age (Vital et al., 1989). Part of the function of the neurocentral cartilage is to ensure growth of the posterior arch of the vertebrae. Early fusion of the neurocentral synchondrosis has been implicated in the development of scoliosis (Vital et al., 1989). Scoliosis is discussed in more detail in Chapter 6.
During development the vertebral bodies may appear to be wedge shaped, narrower anteriorly than posteriorly. This can give the appearance of a compression fracture (Fesmire and Luten, 1989). Wedging that occurs in several consecutive vertebrae is seen as an indication of a normal variant. However, a compression fracture of the wedge-shaped vertebra must be considered if it occurs at only one level and the vertebrae above and below are more rectangular in appearance.
Secondary Ossification Centers.
Five secondary centers of ossification appear in the vertebral column between the ages of 10 and 13 (see Fig. 12-11). One secondary center of ossification is located on each of the cartilaginous end plates of a typical vertebral body. These centers are known as the anular apophyses or ring apophyses (Williams et al., 1995). A secondary center of ossification also is found on the tips of each of the transverse processes, and another is located on the tip of the single spinous process. The centers on the transverse processes and spinous process enable the rapid growth of these processes that occurs during adolescence.
The two centers of ossification associated with the peripheral rim of the upper and lower surfaces of the vertebral bodies (anular apophyses) do not help with the longitudinal growth of the vertebral bodies and for this reason are frequently termed ring apophyses (Theil, Clements, and Cassidy, 1992; Bogduk, 1997). These centers incorporate the outer layers of the anulus fibrosus (Fardon, 1988), which explains the bony attachment of the outer layers of the anulus, whereas the more central layers are attached to the cartilage of the vertebral end plates (Bogduk, 1997).
All of the secondary ossification centers listed previously fuse with the remainder of the vertebrae between the ages of 14 and 25 (Williams et al., 1995; Bogduk, 1997), and no further growth can occur after their fusion. These centers can be mistaken as sites of fracture before they have fused.
Fully Developed Vertebral Column.
The first accurate description of the number of movable vertebrae in the fully developed spine was that of Galen between 100 and 200 AD (Shapiro, 1990). However, perhaps because of the many anatomic errors made by Galen in other areas, controversy ensued over the precise number of vertebrae until the publication of Vesalius’ De Humani Corporis Fabrica in 1543 (Shapiro, 1990). This publication showed that the human vertebral column develops into 24 vertebrae (Fig. 2-1), which are divided into 7 cervical, 12 thoracic, and 5 lumbar vertebrae (expressed as C1-7, T1-12, and L1-5). The L5 vertebra rests on the bony sacrum (made of five fused segments). The coccyx (three to five fused segments) is suspended from the sacrum. All of these bones are joined by means of a series of approximately 136 joints (including the joints between vertebrae and ribs) to form the vertebral column.
CURVES OF THE SPINE
The spine develops four anterior to posterior curves, two kyphoses and two lordoses. (See introduction of text for further clarification of the terms lordosis and kyphosis.) Kyphoses are curves that are concave anteriorly, and lordoses are curves that are concave posteriorly. The two primary curves are the kyphoses. These include the thoracic and pelvic curvatures (see Fig. 2-1). They are called primary curves because they are seen from the earliest stages of fetal development. The thoracic curve extends from T2 to T12 and is created by the larger superior to inferior dimensions of the posterior portion of the thoracic vertebrae (see Chapter 6). The pelvic curve extends from the lumbosacral articulation throughout the sacrum to the tip of the coccyx. The concavity of the pelvic curve faces anteriorly and inferiorly, and is also caused by the greater superior to inferior dimensions of the posterior portion of the sacral segments.
The two secondary curves are the cervical lordosis and lumbar lordosis (see Fig. 2-1). These curves are known as secondary or compensatory curves because, even though they can be detected during fetal development, they do not become apparent until the postnatal period. The cervical lordosis begins late in intrauterine life but becomes apparent when an infant begins to lift his or her head from the prone position (≈3 to 4 months after birth). This forces the cervical spine into a lordotic curve. The cervical lordosis is further accentuated when the small child begins to sit upright and stabilizes his or her head while looking around in the seated position. This occurs at approximately 9 months of age. In the adult, the cervical curve is maintained by the larger superior to inferior dimensions of the anterior portion of the intervertebral discs. Because this curve is primarily created by the pliable intervertebral discs, traction of the cervical region reduces the cervical lordosis, whereas traction to the thoracic region has little effect on the thoracic kyphosis, because the thoracic curve is primarily created by the shape of the vertebrae. Further details of the cervical curvature are given in Chapter 5.
The action of the erector spinae muscles (see Chapter 4), pulling the lumbar spine erect to achieve the position necessary for walking, creates the posterior concavity known as the lumbar lordosis (see Fig. 2-1). Therefore the lumbar lordosis develops approximately 10 to 18 months after birth as the infant begins to walk upright. The lumbar lordosis extends from T12 to the lumbosacral articulation and is more pronounced in females than males. The region between L3 and the lumbosacral angle is more prominently lordotic than the region from T12 to L2. After infancy, the lumbar lordosis is maintained by a combination of the shape of the intervertebral discs and the shape of the vertebral bodies. Each of these structures is taller anteriorly than posteriorly in the lumbar region of the spine. Therefore the lumbar lordosis is reduced when traction forces are applied to it, but the reduction is less than that found during traction of the cervical region.
The lumbar lordosis and thoracic kyphosis both increase from the supine to the standing position (Wood et al., 1996). In addition, the cervical lordosis has been found to compensate for the variations in lumbar lordosis that occur during changes in position and during normal motion. For example, lumbar lordosis increases during sitting in the erect position and cervical lordosis decreases during this activity. Lumbar lordosis decreases during lumbar forward flexion and cervical lordosis increases during lumbar flexion, and the opposite occurs during lumbar extension (Black, McClure, and Polansky, 1996).
The kyphoses and lordoses of the spine, along with the intervertebral discs, help to absorb the loads applied to the spine. These loads include the weight of the trunk, along with loads applied through the lower extremities during walking, running, and jumping. In addition, loads are applied by carrying objects with the upper extrem ities, the pull of spinal muscles, and the wide variety of movements that normally occur in the spine. The spinal curves, acting with the intervertebral discs and vertebral bodies, dissipate the increased loads that would occur if the spine were shaped like a straight column. Yet even with these safeguards, the vertebrae can be fractured as a result of falling and landing on the feet or buttocks, objects falling onto the head, or diving and landing on the head. Such injuries usually compress the vertebral bodies. Cervical compression usually occurs between C4 to C6 (Foreman and Croft, 1988). When the force comes from below, T9 through L2 are the most commonly affected through compression. Flexion injuries also can result in a compression fracture of vertebral bodies. Again, C4 through C6 are the most commonly affected in the cervical region, whereas T5 and T6 and the upper lumbar vertebrae usually are affected in the thoracic and lumbar regions (White and Panjabi, 1990).
ANATOMY OF A TYPICAL VERTEBRA
A typical vertebra can be divided into two basic regions, a vertebral body and vertebral arch (also called the posterior arch or dorsal arch). The bone in both regions is composed of an outer layer of compact bone and a core of trabecular bone, also known as cancellous, or spongy, bone (Fig. 2-2). The cancellous bone is composed of myriad spicules of bone, known as trabeculae (singular, trabecula). The trabeculae are oriented parallel to the lines of greatest stress (Skedros, Mason, and Bloebaum, 1994; Skedros et al., 1994). Smit, Odgaard, and Schneider (1997) found that the trabecular architecture of the lumbar vertebral bodies was ideal for the loads placed on the spine during axial compression (loads placed on the vertebral bodies from above; for example, to resist gravity) and walking. That is, not only were the trabeculae arranged to withstand axial compression, but they also were quite strong where the pedicles of the posterior arch met the vertebral bodies. This latter finding is consistent with the transfer of loads from the articular processes of the posterior arch to the vertebral bodies during rotational movements in the horizontal plane, and anterior to posterior (“shearing”) movements (most closely associated with walking).
The shell of compact bone is thin on the discal surfaces of the vertebral body and is thicker in the vertebral arch and its processes. The outer compact bone is covered by a thin layer of periosteum that is innervated by nerve endings, which transmit both nociception and proprioception (Edgar and Ghadially, 1976). The outer compact bone also contains many small foramina to allow passage for numerous veins and nutrient arteries. The trabecular interior of a vertebra contains red marrow and one or two large canals for the basivertebral vein(s).
The density of bone in the vertebrae varies from individual to individual but seems to increase significantly in most people during puberty and reaches a peak during the mid-twenties, when closure of the growth plates of the secondary centers of ossification occurs (Gilsanz et al., 1988). A decrease in bone mineral density to below normal limits is known as osteoporosis. Osteoporosis also is accompanied by a rearrangement of the trabeculae within the spongy bone (Feltrin et al., 2001). This condition is of particular clinical relevance in the spine because of the weight-bearing function of this region. A decrease in bone mineral density and rearrangement of trabeculae leads to a loss of elasticity in the bone and an increase in bone fragility. These changes, in turn, increase the likelihood of vertebral fracture (Mosekilde and Mosekilde, 1990; Feltrin et al., 2001). Osteoporosis has been associated with aging (Mosekilde and Mosekilde, 1990) and particularly with menopause (Ribot et al., 1988). Ribot et al. (1988) found that spinal bone density in French women remained stable in the young adult years and in women over 70 years of age. An average rate of apparent bone loss of approximately 1% per year was found between the ages of 45 and 65. This represented approximately 75% of the total bone loss occurring within the individuals of their sample population (510 women). Ribot et al. (1988) also found that the bone mineral density in their population of French women appeared to be between 5% and 10% lower than reported values in the United States. Mosekilde and Mosekilde (1990), studying the L2 and L3 vertebrae, found relatively few sex-related differences in vertebral body density. However, Mosekilde (1989) did find a sex-related difference in vertebral trabecular architecture with age. Consistent with the findings of Ribot et al. (1988), Mosekilde (1989) discovered that in both sexes bone density diminished by 35% to 40% from 20 to 80 years of age. She also found that the trabecular center (cancellous bone) of the vertebral body lost more bone mass than the outer cortical rim.
The regions of the vertebral body and vertebral arch are discussed separately in the following sections of this chapter. Elaboration on each component of the vertebra, with special emphasis placed on the characteristics unique to each region of the vertebral column, is included in the chapters on the cervical, thoracic, and lumbar regions of the spine (Chapters 5 through 7). In addition, Table 2-1 compares and contrasts the different parts of cervical, thoracic, and lumbar vertebrae.
Vertebral Body
The vertebral body (Fig. 2-3) is the large anterior portion of a vertebra that acts to support the weight of the human frame. Each vertebral body is designed to provide the greatest amount of strength with the least amount of bone mass (Feltrin et al., 2001). The vertebral bodies are connected to one another by fibrocartilaginous intervertebral discs, and when the bodies are combined with their intervening discs, they create a flexible column or pillar that supports the weight of the trunk and head. The vertebral bodies also must be able to withstand additional forces from contraction of the axial and proximal limb muscles. The bodies are cylindric in shape and have unique characteristics in each named region of the spine. The transverse diameter of the vertebral bodies increases from C2 to L3. This probably results from the fact that each successive vertebral body carries a slightly greater load. There is variation in the width of the last two lumbar vertebrae, but the width steadily diminishes from the first sacral segment to the apex (inferior tip) of the coccyx.
Vertical trabeculae predominate in the vertebral bodies. The vertical trabeculae are supported by horizontal trabeculae that function much like the struts or support beams in the frame of a building. Animal studies have shown that both the vertical and horizontal trabeculae of a vertebral body increase in number after prolonged (weeks) and increased loading by superior-inferior compression (Issever et al., 2003).
Bone mineral density can vary significantly from one vertebra to another (Curylo et al., 1996). Although determining the presence or absence of osteoporosis by means of x-ray bone densitometry to measure bone mineral density is reliable, fractal analysis of the trabecular pattern within vertebral bodies as imaged by computed tomography (CT) also shows promise (Feltrin et al., 2001).
The vertebral bodies have been found to change (remodel) after degeneration of the intervertebral discs, by adding bone to the region adjacent to the intervertebral disc. This addition of bone is known as subchondral sclerosis, and allows the vertebral bodies to more effectively absorb the additional compressive loads received by the vertebral bodies after intervertebral disc degeneration (Moore et al., 1996).
Mosekilde and Mosekilde (1990) found that the cross-sectional area of vertebral bodies is larger in men than in women. They also found that the cross-sectional area of the vertebral bodies increases with age in men, but no similar finding was discovered in women.
The superior and inferior surfaces of vertebral bodies range from flat, but not parallel (Williams et al., 1995), to interlocking (see Chapter 5). A raised, smooth region around the edge of the vertebral body is formed by the anular apophysis. The vertebral body is rougher inside the anular apophysis.
Most vertebral bodies are concave posteriorly (in the transverse plane), where they help to form the vertebral foramina. Small foramina for arteries and veins appear on the front and sides of the vertebral bodies. Posteriorly there are small arterial foramina and one or two large, centrally placed foramina for the exiting basivertebral vein(s) (Williams et al., 1995).
A series of relatively large arteries pierce the center of the vertebral bodies along their entire circumference (Fig. 2-4). On entering a vertebral body, these large nutrient arteries form a dense plexus of arteries within the central horizontal plane of the vertebral body. From this central plexus, many small branches ascend and descend to reach the superior and inferior margins of the vertebral bodies; these margins are adjacent to the cartilaginous end plates of the intervertebral discs.
Large numbers of small veins drain the superior and inferior margins of the vertebral bodies. These very small veins enter into large tributaries that are oriented in the horizontal plane very close to each superior and inferior vertebral margin. These large tributaries have been called the horizontal subarticular collecting vein system (Crock and Yoshizawa, 1976). Branches of the horizontal subarticular collecting vein system, in turn, drain into large vertically oriented channels that course toward the central horizontal plane of the vertebral body, where a dense venous network is formed. The dense network is drained by the basivertebral vein (occasionally there are two basivertebral veins in the same vertebral body). The subarticular collecting vein system also sends small tributaries laterally. These small tributaries leave the vertebral body and drain into veins of the external vertebral venous plexus (see later information).
Of clinical interest are the findings of Esses and Monro (1992) who found that long-term intraosseous hypertension within the vessels of the vertebral bodies is associated with an increase of pain and severity of osteoarthritis.
Occasionally a vertebral body compression fracture occurs some time (days to years) after an individual suffers trauma to the spine. This condition is known as “delayed posttraumatic vertebral collapse,” or Kümmell disease, and is probably the result of damage to the nutrient arteries of the vertebral body during the original injury. Damage to the nutrient arteries then leads to necrosis (ischemic necrosis) of the vertebral body and subsequent vertebral collapse (Van Eenenaam and El-Khoury, 1993).
Osteophytes of the vertebral bodies are protrusions of the superior or inferior aspects of the vertebral bodies that are composed of compact bone and extend toward the adjacent intervertebral disc and vertebral body. Anterior osteophytes of the vertebral bodies generally are more common than posterior ones and usually are larger. A large proportion of vertebral columns have osteophytes by the second decade of life, and by the fourth decade osteophytes are present in almost 100% of vertebral columns. The size of the osteophytes increases with age. There is no significant difference between osteophyte formation on the anterior aspect of the vertebral bodies and race; however, males have more anterior osteophytes than females. Osteophytes on the posterior aspect of the vertebral bodies are most common in the lower cervical and lower lumbar regions and are more common in white than in black males and females. No significant difference exists in the prevalence of posterior osteophytes between males and females of the same racial background (Nathan, 1962).
Osteophytes develop slightly earlier in life in the thoracic and lumbar regions than in the cervical and sacral regions. However, in the fifth decade cervical osteophytes develop more rapidly than in the other regions of the spine, and by the seventh decade the incidence is roughly equal among cervical, thoracic, and lumbar osteophytes; osteophytes of the sacral region (only found on the first sacral segment) are the least common (Nathan, 1962).
Anterior osteophytes can result in complete interbody fusion. Such fusion is most common in the mid- to lower-thoracic region and in the lower-cervical region; however, fusion is extremely rare between C7 and T1 and between L5 and the first sacral segment (Nathan, 1962).
Osteophytes are much less common in the region of a vertebral body in contact with the aorta, and they usually develop in the region of the vertebral body that receives the greatest compressive loads during normal stance or common movements. For example, osteophytes tend to develop on the concave side of the normal curves of the spine. Anterior osteophytes, which generally are the most numerous, are most common in the thoracic region, and posterior osteophytes are most common in the cervical and lumbar regions of the spine (Nathan, 1962).
Bony End Plates.
The ring apophyses, also known as the ring apophyses, are secondary centers of ossification that develop along the periphery of the superior and inferior aspects of the vertebral bodies before puberty (see Chapter 12). These regions fuse with the remainder of the vertebral bodies usually by the age of 25 years. Some authors refer to the superior- and inferior-most regions of the vertebral body, including the area associated with the superior and inferior ring apophyses (both before and after their fusion with the remainder of the vertebral body), as the vertebral end plates. However, this terminology is confusing because the vertebral end plates (also known as the cartilaginous end plates) refer to the parts of each intervertebral disc that are found superior and inferior to the nucleus pulposus and anulus fibrosus. Therefore the term “bony end plate” is used in this text to describe the superior- and inferior-most regions of the vertebral bodies. During the time of puberty these regions are also associated with the ring apophyses, and the term bony end plate applies to the region of the ring apophyses as well, both before and after their fusion with the remainder of the vertebral bodies. The term vertebral end plate, or cartilaginous end plate, is used in this text to refer to the superior and inferior aspects of each intervertebral disc.
Between 6 months and 2.5 years of age the mottled appearance of the central bony end plate disappears (as do the blood vessels), and the end plate retains this smoother appearance for the remainder of the life of the vertebra. However, between 6 months and 25 years of age the peripheral margins of the end plates become prominently scalloped, showing prominent ridges and sulci. This scalloping results in a denticulate, or toothlike, appearance along the vertebral margins, having an appearance similar to that of the outer margins of the epiphyseal plates of other bones of the body. The scalloping of the bony end plate is variable from one vertebra to the next and is most prominent in the lower thoracic and upper lumbar regions, and less pronounced in the cervical and thoracic regions. The scalloping is thought to increase stability during the application of shear forces to the spine (forces that tend to slide one vertebra over the vertebra immediately below). Resistance to shear forces also explains why the scalloping is less prominent in the thoracic and cervical regions, where the ribs and uncinate processes (see Chapter 5), respectively, resist shear forces in these areas. The ridges and sulci of the bony end plates become more prominent until approximately 12 to 25 years of age when the bone of the anular apophysis is laid down over them, creating an enlarging smooth ridge of bone that follows the peripheral margins of the superior and inferior surfaces of the vertebral bodies (Edelson and Nathan, 1988).
Beginning in the latter aspect of the third decade, osteophytes develop on the vertebral bodies, usually just adjacent to the bony end plate. That is, an osteophyte usually spares the bony end plate (there is usually a distinct sulcus between each osteophyte and the related bony end plate). The osteophytes then arch across the bony end plate, extending toward the adjacent vertebra (Edelson and Nathan, 1988).
Osteoporotic changes also can occur in the bony end plate. These changes usually begin toward the end of the fifth decade and progress until death. Osteoporotic changes in the bony end plates take on the appearance of lytic, or “punched out” areas of the bone (Edelson and Nathan, 1988).
Vertebral Arch
The vertebral (posterior) arch has several unique structures (see Fig. 2-3). These include the pedicles, laminae, superior articular, inferior articular, transverse, and spinous processes. Each of these subdivisions of the vertebral arch is discussed separately in the following sections.
Pedicles.
The pedicles (see Fig. 2-3) create the narrow anterior portions of the vertebral arch. They are short, thick, and rounded and attach to the posterior and lateral aspects of the vertebral body. They also are placed superior to the midpoint of a vertebral body. Because the pedicles are smaller than the vertebral bodies, a groove, or vertebral notch, is formed above and below the pedicles. These are known as the superior and inferior vertebral notches, respectively. The superior vertebral notch is more shallow and smaller than the inferior vertebral notch.
The percentage of compact bone surrounding the inner cancellous bone of the pedicles varies from one region of the spine to another and seems to depend on the amount of motion that occurs at the given region (Pal et al., 1988). More compact, stronger bone is found in regions with more motion. Therefore the pedicles of the middle cervical and upper lumbar regions contain more compact bone than the relatively immobile thoracic region. The thoracic pedicles are made primarily of cancellous bone (Pal et al., 1988).
There are significant differences in the relative size of various parts of vertebrae among various ethnic populations, with those from Western populations generally having larger structures than those from Asia. This is true for the pedicles (Chadha et al., 2003).
Laminae.
The laminae (singular, lamina) are continuous with the pedicles. They are flattened from anterior to posterior and form the broad posterior portion of the vertebral arch (see Fig. 2-3). They curve posteromedially to unite with the spinous process, completing the vertebral foramen. Xu et al. (1999) performed a detailed morphometric study of the laminae of the entire vertebral column. They concluded that, generally speaking, the laminae of males are slightly larger than those of females. The laminae generally increase in height from C4, which are the shortest (10.4 + 1.1 mm), to T11, which are the tallest (25.1 + 2.5 mm). The height of the laminae then begin to decrease slowly from T12 to L4, and then more markedly at L5. However, the laminae are widest at L5 (15.7 + 2.0 mm) and narrowest at T4 (5.8 + 0.8 mm). The cervical laminae are wide (rivaling those of L5), the thoracic laminae (with the exception of T11 and T12) are narrow, and the width steadily increases from T11 to L5. The laminae are thickest at T2 (5.0 + 0.2 mm) and least thick at C5 (1.9 + 0.6 mm), with the thickness of the laminae decreasing from the upper to the lower thoracic regions. The lower cervical laminae are the least thick of the vertebral column, and the lumbar laminae are of intermediate thickness (Xu et al., 1999).
Spinous Process.
The spinous process (spine) of each vertebra (see Fig. 2-3) projects posteriorly and often inferiorly from the laminae. The size, shape, and direction of this process vary greatly from one region of the vertebral column to the next (see individual regions). A spinous process also may normally deviate to the left or right of the midline, and this can be a source of confusion in clinical practice. Therefore a deviated spinous process seen on x-ray film or palpated during a physical examination frequently is not associated with a fracture of the spinous process or a malposition of the entire vertebra.
The spinous processes throughout the spine function as a series of levers both for muscles of posture and muscles of active movement (Williams et al., 1995). Most of the muscles that attach to the spinous processes act to extend the vertebral column. Some muscles attaching to the spinous processes also rotate the vertebrae to which they attach.
The spinous process of a specific vertebra frequently can be identified by its relationship to other palpable landmarks of the back. Chapter 1 provides a detailed account of the relationship between the spinous processes and other anatomic structures.
Vertebral Foramen and the Vertebral Canal.
The vertebral foramen is the opening within each vertebra that is bounded by the structures discussed thus far. Therefore the vertebral body, the left and right pedicles, the left and right laminae, and the spinous process form the borders of the vertebral foramen in a typical vertebra (see Fig. 2-3). The size and shape of the vertebral foramina vary from one region of the spine to the next and even from one vertebra to the next. The vertebral canal is the composite of all of the vertebral foramina. This region houses the spinal cord, nerve roots, meninges, and many vessels. The vertebral canal is discussed in more detail later in this chapter.
Transverse Processes.
The transverse processes project laterally from the junction of the pedicle and lamina (pediculolaminar junction) (see Fig. 2-3). Like the spinous processes, their exact direction varies considerably from one region of the spine to the next. The transverse processes of typical cervical vertebrae project obliquely anteriorly between the sagittal and coronal planes and are located anterior to the articular processes and lateral to the pedicles. The left and right cervical transverse processes are separated from those of the vertebrae above and below by successive intervertebral foramina. The thoracic transverse processes are different and project obliquely posteriorly and are located behind the articular processes, pedicles, and intervertebral foramina (see Fig. 6-1). They also articulate with the ribs. The lumbar transverse processes (see Fig. 7-1) lie in front of the lumbar articular processes and posterior to the pedicles and intervertebral foramina.
Each transverse process is composed of the “true” transverse process (diapophysis) and a costal element. Each costal element (pleurapophysis) develops as part of the neural arch (see Fig. 12-13). The costal elements of the thoracic region develop into ribs. Elsewhere the costal elements are incorporated with the diapophysis and help to form the transverse process of the fully developed vertebra. The cervical costal elements are composed primarily of the anterior tubercle but also include the intertubercular lamella and a part of the posterior tubercle. The lumbar costal elements are the anterior aspects of the transverse processes, and the left and right sacral alae represent the costal processes of the sacrum. The cervical and lumbar costal processes occasionally may develop into ribs. This occurs most frequently in the lower cervical and upper lumbar regions. These extra ribs may be a cause of discomfort in some individuals. This is particularly true of cervical ribs (see Chapter 5).
Superior Articular Processes.
Like the transverse processes, the superior articular processes (zygapophyses) and facets also arise from the pediculolaminar junction (see Fig. 2-3). The left and right superior articular processes project superiorly, and the articular surface (facet) of each articular process faces posteriorly, although the precise direction varies from posteromedial in the cervical and lumbar regions to posterolateral in the thoracic region. (The superior and inferior articular facets are discussed in more detail later in this chapter under Zygapophysial Joints.)
Inferior Articular Processes.
The left and right inferior articular processes (zygapophyses) and facets project inferiorly from the pediculolaminar junction, and the articular surface (facet) faces anteriorly (see Fig. 2-3). Again, the precise direction in which they face varies from anterolateral (cervical region) to anteromedial (thoracic and lumbar regions).
Functional Components of a Typical Vertebra
Each region of a typical vertebra is related to one or more of the functions of the vertebral column mentioned at the beginning of this chapter (support, protection of the spinal cord and spinal nerve roots, and movement) (Fig. 2-5). In general, the vertebral bodies help with support, whereas the pedicles and laminae protect the spinal cord. The superior and inferior articular processes help determine spinal movement by the facing of their facets. The transverse and spinous processes aid movement by acting as lever arms on which the muscles of the spine act.
The posterior arches also function to support and transfer weight (Pal et al., 1988), and the articular processes of the cervical region form two distinct pillars (left and right) that bear weight. In addition, the laminae of C2, C7, and the upper thoracic region (T1 and T2) help to support weight. Therefore a laminectomy at these levels results in marked cervical instability (Pal et al., 1988), whereas a laminectomy from C3 to C6 is relatively safe.
The pedicles also act to transfer weight from the posterior arch to the vertebral body, and vice versa, in the cervical region (Pal et al., 1988), but only from the posterior arch to the vertebral bodies in the thoracic region. The role of the pedicles in the transfer of loads is yet to be completely determined in the upper lumbar region, but the trabecular pattern of the L4 and L5 pedicles seems to indicate that the majority of load may be transferred from the vertebral bodies to the region of the posterior arch. This is discussed in further detail in Chapter 7, which is devoted to the lumbar spine.
ZYGAPOPHYSIAL JOINTS
The articulating surface of each superior and inferior articular process (zygapophysis) is covered with a 1- to 2-mm-thick layer of hyaline cartilage. This hyaline-lined portion of a superior and inferior articular process is known as the articular facet. The junction found between the superior and inferior articular facets on one side of two adjacent vertebrae is known as a zygapophysial joint. Therefore a left and right Z joint are between each pair of vertebrae. Figure 2-6, A shows the Z joints of the cervical, thoracic, and lumbar regions. These joints also are called facet joints or interlaminar joints (Giles, 1992). The Z joints (Fig. 2-6, B to D) are classified as synovial (diarthrodial), planar joints. They are rather small joints, and although they allow motion to occur, they are perhaps more important in their ability to determine the direction and limitations of movement that can occur between vertebrae. In addition, the Z joints (more specifically, the articular processes) help to carry the loads placed on the spine, particularly during extension and rotation (Schultz et al., 1973). The Z joints are of added interest to those who treat spinal conditions because, as is the case with any joint, loss of motion or aberrant motion may be a primary source of pain (Paris, 1983). In fact, Dreyer and Dreyfuss (1996) estimate that 15% to 40% of chronic low back pain is related to the Z joints.
Each Z joint is surrounded posterolaterally by a capsule. The outer capsule and inner layers of the capsule differ significantly in make-up; this is possibly unique to Z joints (Yamashita et al., 1996). The capsule consists of an outer layer of dense fibroelastic connective tissue, a vascular central layer made up of areolar tissue and loose connective tissue, and an inner layer consisting of a synovial membrane (Giles and Taylor, 1987). Figure 2-6, B, shows the previously listed regions of the capsule. The anterior and medial aspects of the Z joint are covered by the ligamentum flavum. The synovial membrane lines the articular capsule, the ligamentum flavum (Xu et al., 1991), and the synovial joint folds (see the following), but not the hyaline articular cartilage that covers the joint surfaces of the articular processes (Giles, 1992).
The Z joint capsules throughout the vertebral column are thought to do little to limit motion (Onan, Heggeness, and Hipp, 1998), although the capsules probably help to stabilize the Z joints during motions (Boszczyk et al., 2001). Generally, the Z joint capsules are relatively thin and loose and are attached to the margins of the opposed superior and inferior articular facets of the adjacent vertebrae (Williams et al., 1995). Superior and inferior external protrusions of the joint capsules, known as recesses, bulge out from the joint and are filled with adipose tissue. The inferior recess is larger than the superior one (Jeffries, 1988). The capsules are longer and looser in the cervical region than in the lumbar and thoracic regions.
Innervation of the Zygapophysial Joints
The Z joint capsule receives a significant sensory inneration (Cavanaugh, Kallakuri, and Özaktay, 1995; Vandenabeele, Creemers, and Lambrichts, 1996). Ahmed et al. (1993) found both sensory and autonomic fibers in the synovial layer of the Z joint capsules of rats. They also found evidence of nociceptive innervation in the ligamentum flavum proper (that portion of the ligamentum flavum adjacent to the Z joint). The authors concluded that both the sensory and autonomic innervations could play a collaborative role in the pathophysiology of Z joint pain, inflammation, and inflammatory joint disease.
The sensory nerve supply to each Z joint (Fig. 2-7) is derived from the medial branch of the posterior primary division (dorsal ramus) at the level of the joint, and each joint also receives a branch from the medial branch of the posterior primary division of the level above and the level below (Jeffries, 1988). This multilevel innervation is probably one reason why pain from a Z joint frequently has a very broad referral pattern (Jeffries, 1988). Chapter 11 deals with the phenomenon of referred pain in more detail.
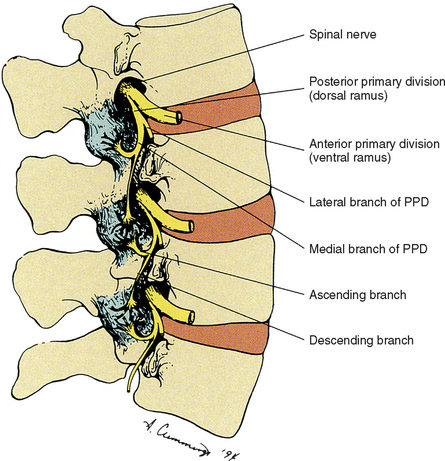
FIG. 2-7 Innervation of the Z joints. Each spinal nerve divides into a medial and lateral branch. The medial branch has an ascending division, which supplies the Z joint at the same level, and a descending division, which supplies the Z joint immediately below. Jeffries (1988) states that each medial branch also sends a branch to the Z joint of the level above (not shown in illustration).
The medial branches of the posterior primary divisions innervating a Z joint terminate as one of three types of sensory receptors: free nerve endings (nociceptive), complex unencapsulated nerve endings, and encapsulated nerve endings (Yamashita et al., 1990; Beaman et al., 1993; Cavanaugh, Kallakuri, and Özaktay, 1995). The latter two types are thought to be associated with proprioceptive sense and the modulation of protective muscular reflexes. The free nerve endings are associated with nociception (i.e., signaling potential or real tissue damage). The ultrastructure of these receptors has been described (Vandenabeele et al., 1997; McLain and Pickar, 1998).
In addition, Wyke (1985) categorized the types of sensory receptors in Z joints by their function. These categories are as follows:
Wyke (1985) asserts that type I and II receptors have a pain suppressive effect (a Melzack and Wall gate control type of mechanism). He also states that there is a reflexogenic effect created by type I and II fibers that causes a normalization of muscle activity on both sides of the spinal column when stimulated. This reflexogenic effect is thought to occur at the level of the site of stimulation, as well as at the levels above and below. Of possible interest is the fact that Isherwood and Antoun (1980) found similar nerve endings within the interspinous and supraspinous ligaments and the ligamentum flavum. These ligaments are discussed in Chapters 5 and 6 on the cervical and thoracic regions.
Innervation by mechanoreceptors is denser in the cervical Z joint capsules than in those of the thoracic and lumbar regions (McLain, 1994; McLain and Pickar, 1998). This may be because the increased mobility of the cervical region may require more proprioceptive input to ensure smooth and accurate head movement and positioning, and also to help prevent injury from inappropriate motions or muscle responses to sudden movements. Innervation by free nerve endings associated with nociception is abundant in all regions of the spine (McLain and Pickar, 1998).
Beaman et al. (1993) found nerves that stained with substance P (associated with pain) in the bone underlying the articular facets of the articular processes (subchondral bone) from specimens of Z joints taken during surgical procedures of patients with low back pain and accompanying degeneration of the Z joints, but not in control specimens. This indicates that the subchondral bone may be an additional source of pain in individuals with arthritis of the spine (including degenerative joint disease, also known as osteoarthritis, or common degenerative arthritis) or with injury to the spine. Because degeneration of the spine can result in an increase in the loads placed on the Z joints by 3% to 47%, depending on the severity of the degeneration, the presence of nociceptive (pain) nerve endings in the subchondral bone of the Z joint articular facets indicates that the subchondral bone in this region may play an active role in back pain. The combined innervation of the Z joint capsules and subchondral bone provides further strong evidence implicating the Z joints as an important source of back pain in many individuals.
Zygapophysial Joint Synovial Folds
Z joint synovial folds are synovium-lined extensions of the capsule that protrude into the joint space to cover part of the hyaline cartilage. The synovial folds vary in size and shape in the different regions of the spine. Figure 2-8 shows a photomicrograph by Singer, Giles, and Day (1990) demonstrating a large Z joint synovial fold.
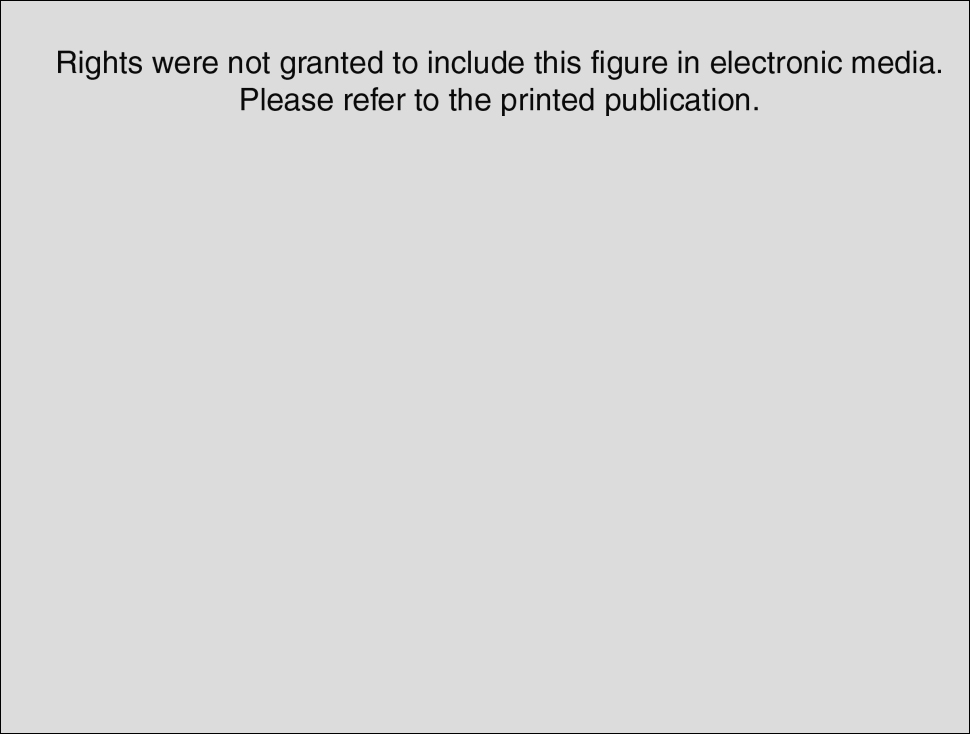
FIG. 2-8 A fibrous synovial fold is shown protruding between the articular surfaces of a Z joint.
(From Singer K, Giles D, & Day R. [1990]. Intra-articular synovial folds of thoracolumbar junction zygapophyseal joints. Anat Rec, 226, 147-152.)
Kos in 1969 described the typical intraarticular fold (meniscus) (Fig. 2-9) as being attached to the capsule by loose connective tissue. Synovial tissue and blood vessels were distal to the attachment, followed by dense connective tissue (Bogduk and Engel, 1984).
Engel and Bogduk in 1982 reported on a study of 82 lumbar Z joints. They found at least one intraarticular fold within each joint. The intraarticular structures were categorized into three types. The first was described as a connective tissue rim found running along the most peripheral edge of the entire joint. This connective tissue rim was lined by a synovial membrane. The second type of meniscus was described as an adipose tissue pad, and the third type was identified as a distinct, well-defined, fibroadipose meniscoid. This latter type of meniscus usually was found entering the joint from either the superior or inferior pole or both poles of the joint.
Giles and Taylor (1987) studied 30 Z joints, all of which were found to have menisci. The menisci were renamed zygapophysial joint synovial folds because of their histologic make-up. Free nerve endings were found within the folds, and the nerve endings met the criteria necessary for classification as pain receptors (nociceptors). That is, they were distant from blood vessels and were of proper diameter (0.6 to 12 μ). Therefore the synovial folds (menisci) themselves were found to be pain sensitive. This meant that if the Z joint synovial fold became compressed by, or trapped between, the articular facets making up the Z joint, back pain could result (see Fig. 2-9). Other investigators have confirmed the presence of sensory fibers in the Z joint synovial folds (Ahmed et al., 1993; Vandenabeele, Creemers, and Lambrichts, 1996).
Zygapophysial Joints as a Source of Back Pain
Various Clinical Approaches to Pain Management.
Damage to the osseous and ligamentous tissues (including the capsule) of a Z joint can result in inflammation, which can cause the release of chemicals that stimulate the nociceptive nerve endings supplying the joint (Cavanaugh, Kallakuri, and Özaktay, 1995; Cavanaugh et al., 1996, 1997). Therefore not surprisingly, the Z joints have been shown to be a source of back pain (Mooney and Robertson, 1976; Lippitt, 1984; Jeffries, 1988; Beaman et al., 1993), and several therapeutic approaches have been designed to treat pain originating from the Z joints. Physical therapy in the form of ice, moist heat, or exercise is used frequently. Acupuncture also has been used. Injection of the Z joints with local anesthetic or corticosteroids is carried out with some frequency, and denervation of the Z joints has been performed by a number of clinicians and researchers (Shealy, 1975). Surgical transection of the posterior primary divisions innervating these joints was the first method used to denervate the joint. This technique has been replaced by radiofrequency neurotomy (Shealy, 1975). Others are not yet convinced that this is the method of choice for treating pain arising from these structures (Lippitt, 1984), especially in light of the fact that damage to the medial branch of the posterior primary division during surgical laminectomy has been linked to prolonged postoperative pain, denervation atrophy of paraspinal back muscles, and functional instability that can extend beyond the segments involved in the surgical procedure (Sihvonen et al., 1993; Boelderl et al., 2002). Spinal adjusting (manipulation) to introduce movement into a Z joint suspected of being hypomobile also has been used frequently to treat pain of Z joint origin. Mooney and Robertson (1976) stated that spinal manipulation may produce therapeutic benefit by relieving the Z joint articular capsule or its synovial lining from chronic reaction to trauma. Such chronic reaction to trauma resulting in Z joint pain includes the catching of a synovial fold between the joint capsule and an articular process and also the entrapment of zygapophysial joint menisci (synovial folds) deep within the Z joint (see Fig. 2-9). Entrapped Z joint menisci may be a direct source of pain because they are supplied by pain-sensitive nerve endings (Giles and Taylor, 1987; Ahmed et al., 1993; Vandenabeele, Creemers, and Lambrichts, 1996). Spinal adjusting (manipulation) separates (gaps) the opposed articular surfaces of the Z joint (Cramer et al., 2000, 2002), and this separation may relieve direct pressure on the meniscus, and also provide traction to the Z joint articular capsule that, by its attachment to the Z joint meniscus, could pull the meniscus peripherally, away from the region of previous entrapment (Kos and Wolf, 1972). Bogduk and Engel (1984) felt that entrapment of a Z joint meniscus would tear it away from its capsular attachment. If this were the case, the nerve endings leading to the synovial fold probably would be torn as well. This could result in transient pain. Bogduk and Engel (1984) also stated that a meniscus that had torn away from its capsular attachment could conceivably result in a loose body being found in the Z joint, similar to those that are sometimes found in the knee. This, they felt, may be amenable to spinal manipulation. However, the frequency with which this scenario actually occurs in clinical practice was questioned (Bogduk and Engel, 1984). Further research is needed to clarify the frequency with which Z joint menisci (synovial folds) actually tear away from their capsular attachments to become loose bodies. Additional study also is needed to determine whether menisci can become entrapped while remaining attached to the capsule and their nerve supply.
Mooney and Robertson (1976) used facet joint injections of local anesthetic and corticosteroids to treat pain arising from the Z joint. They felt that such injections helped to relieve intraarticular adhesions that had been seen to develop during the degenerative phase of progressive back pain. Because hypomobility results in degenerative changes of the Z joints (Cramer et al., 2004), perhaps the removal of this type of adhesion could be another positive effect of Z joint manipulation.
MOVEMENT OF THE SPINE
Movement between two typical adjacent vertebrae is slight, but when the movement between many segments is combined, the result is a great deal of movement. The movements that can occur in the spine include flexion, extension, lateral flexion (side bending), rotation, and circumduction (Fig. 2-10). Circumduction is a combination of flexion, lateral bending, rotation, and extension. The intervertebral discs help to limit the amount of movement that can occur between individual vertebrae. Therefore the thicker intervertebral discs of the cervical and lumbar regions allow for more movement to occur in these regions. In addition, the shape and orientation of the articular facets determine the movements that can occur between two adjacent segments and also limit the amount of movement that can occur between segments. Interestingly, the beginning and middle stages of degeneration (stages I through IV) of the intervertebral discs increase segmental motion, as the thinning discs allow more “joint play” between the segments. However, once end-stage degeneration is reached (stage V), segmental motion decreases. In addition, the early stages of degeneration of the Z joints (stages I through III; accompanied by erosion of the articular hyaline cartilage) increases rotation (axial rotation) of the spine. However, during the later stages of Z joint degeneration, additional bone is added to the subchondral bone subjacent to the Z joint hyaline cartilage articular facets. This process is known as subchondral sclerosis. Once subchondral sclerosis of the Z joints occurs, axial rotation of the involved segments of the spine decreases to below normal levels (Fujiwara et al., 2000).
The specific ranges of motion of the spine are discussed with each vertebral region (see Chapters 5 through 7). However, this section discusses the factors limiting spinal motion and the phenomenon of coupled motion.
The Role of Spinal Ligaments
The ligaments of the spine are discussed from superior to inferior with the region in which they first occur (e.g., ligamentum nuchae and anterior longitudinal ligament with the cervical spine; supraspinous ligament with the thoracic spine). Thereafter, the ligaments are mentioned only when they have unique characteristics in a specific region. The intervertebral disc is covered later in this chapter. However, this section discusses some of the characteristics common to the majority of spinal ligaments. In addition, Tables 2-2 and 2-3 summarize the attachment sites and functions (motions restricted) of the most important ligaments of the spine.
Using nerve tracing techniques in an animal model, Jiang (1997) found that stretching of a spinal ligament resulted in “a barrage of sensory feedback from several spinal cord levels on both sides of the spinal cord.” The sensory information was found to ascend to many higher centers by way of the dorsal columns and spinocerebellar tracts (see Chapter 9). These higher centers included the thalamus and vestibular nuclei. Jiang’s (1997) findings provide provocative evidence that the spinal ligaments, along with the Z joint capsules and the small muscles of the spine (interspinales, intertransversarii, and transversospinalis muscles) play an important role in mechanisms related to spinal proprioception (joint position sense). In addition, Solomonow et al. (1998) found that compression of the supraspinous ligament of cats or electrical stimulation of the same ligament in humans resulted in contraction of the multifidus muscle (see Chapter 4
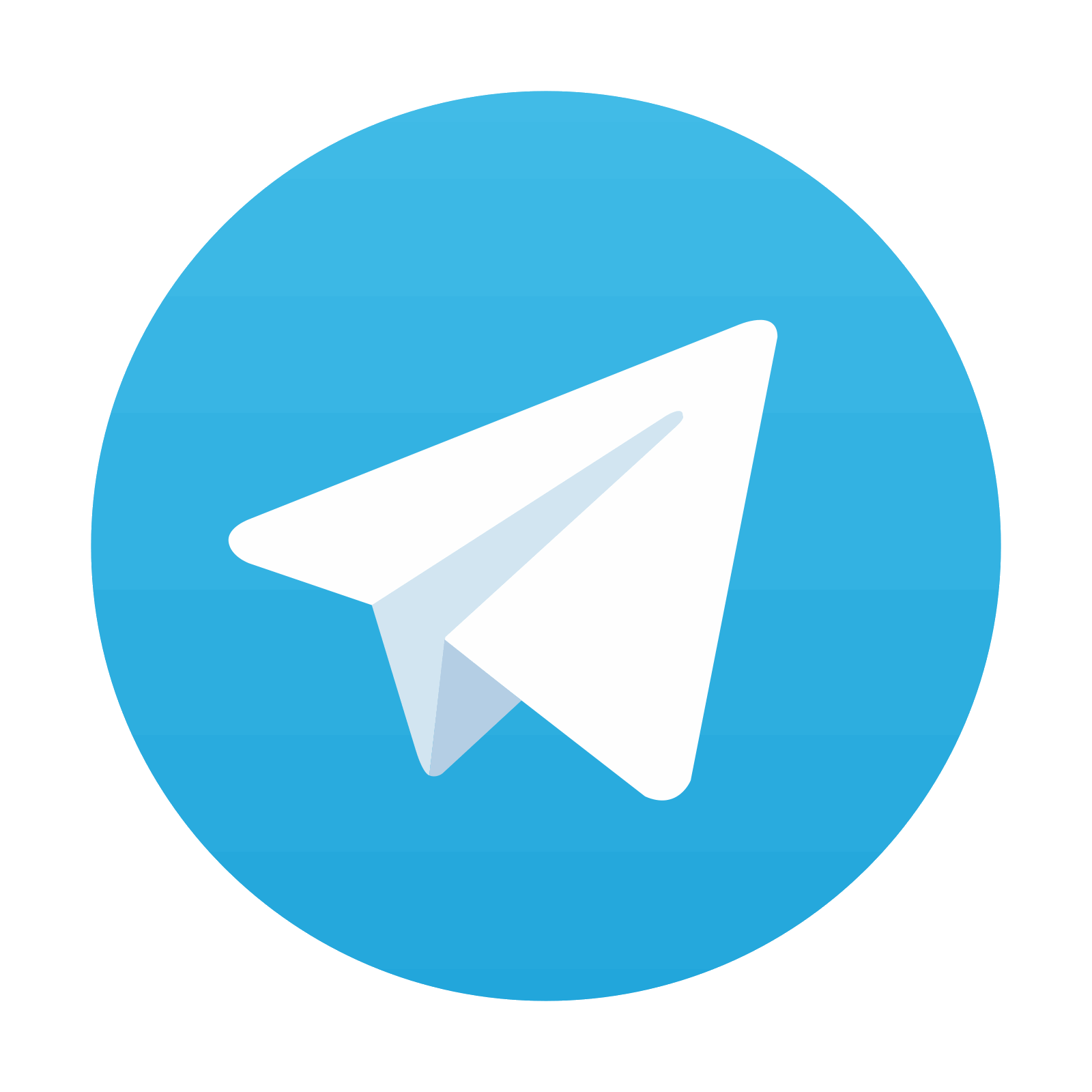
Stay updated, free articles. Join our Telegram channel
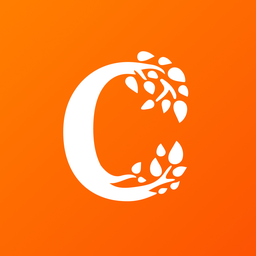
Full access? Get Clinical Tree
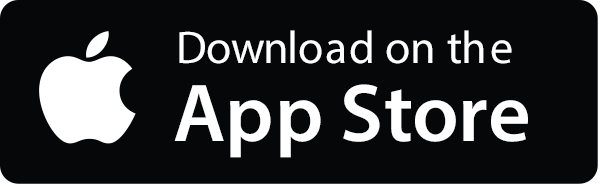
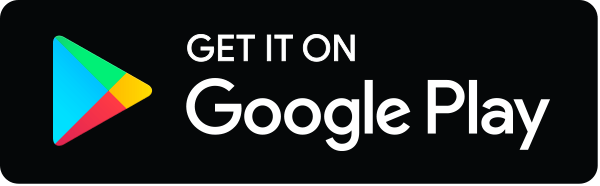