Figure 44-1. Topographic relations of the stomach.
As a first approximation, the lymphatic drainage of the stomach parallels gastric venous return (Fig. 44-3). Lymph from the proximal portion of the stomach along the lesser curvature first drains into superior gastric lymph nodes surrounding the left gastric artery. The distal portion of the lesser curvature drains through suprapyloric nodes. The proximal portion of the greater curvature is supplied by lymphatic vessels that traverse pancreaticosplenic nodes, whereas the antral portion of the greater curvature drains into the subpyloric and omental nodal groups. Secondary drainage from each of these systems eventually traverses nodes at the base of the celiac axis. These discrete anatomic groupings are misleading. The lymphatic drainage of the human stomach, like its blood supply, exhibits extensive intramural ramifications and a number of extramural communications. As a consequence, disease processes that involve the gastric lymphatics often spread intramurally beyond the region of origin and to nodal groups at a distance from the primary lymphatic zone.
The left and right vagal nerves descend parallel to the esophagus within the thorax before forming a periesophageal plexus between the tracheal bifurcation and the diaphragm. From this plexus, two vagal trunks coalesce before passing through the esophageal hiatus of the diaphragm (Fig. 44-4). The left vagal trunk is usually closely applied to the anterior surface of the esophagus, whereas the posterior vagal trunk is often midway between the esophagus and the aorta. The anterior vagus supplies a hepatic division, which passes to the right in the lesser omentum before innervating the liver and biliary tract. The remainder of the anterior vagal fibers parallels the lesser curvature of the stomach, branching to the anterior gastric wall. The posterior vagus nerve branches into the celiac division, which passes to the celiac plexus, and a posterior gastric division, which innervates the posterior gastric wall.
Figure 44-2. Arterial blood supply of the stomach.
Figure 44-3. Lymphatic drainage of the stomach.
Approximately 90% of the fibers in the vagal trunks are afferent, transmitting information from the gastrointestinal tract to the central nervous system (CNS). Parasympathetic afferent fibers are not responsible for the sensation of gastric pain. Only 10% of vagal nerve fibers are motor or secretory efferents. Parasympathetic efferent fibers contained in the vagus originate in the dorsal nucleus of the medulla. Vagal efferent fibers pass without synapse to contact postsynaptic neurons in the gastric wall in the myenteric and submucous plexuses. Secondary neurons directly innervate gastric smooth muscle or epithelial cells. Acetylcholine is the neurotransmitter of primary vagal efferent neurons.
The gastric sympathetic innervation is derived from spinal segments T5 through T10. Sympathetic fibers leave the corresponding spinal nerve roots by way of gray rami communicantes and enter a series of bilateral prevertebral ganglia (Fig. 44-5). From these ganglia, presynaptic fibers pass through the greater splanchnic nerves to the celiac plexus, where they synapse with secondary sympathetic neurons. Postsynaptic sympathetic nerve fibers enter the stomach in association with blood vessels. Afferent sympathetic fibers pass without synapse from the stomach to dorsal spinal roots. Pain of gastroduodenal origin is sensed through afferent fibers of sympathetic origin.
Figure 44-4. Vagal innervation of the stomach.
Figure 44-5. Derivation of gastric sympathetic innervation.
MICROSCOPIC ANATOMY
The glandular portions of the stomach are lined by a simple columnar epithelium composed of surface mucous cells. The luminal surface, visualized by scanning electron microscopy, appears cobblestoned, interrupted at intervals by gastric pits. Opening into the gastric pits are one or more gastric glands that impart functional significance to the gastric mucosa. The mucosa of the human stomach is composed of three distinct types of gastric glands – cardiac, oxyntic, and antral.
In humans, cardiac glands occupy a narrow zone adjacent to the esophagus and mark a transition from the stratified squamous epithelium of the esophagus to the simple columnar epithelium of the stomach. The surface and gastric pit mucous cells of the cardia are not distinguishable from those in other areas of the stomach. Cardiac glands contain mucous and undifferentiated and endocrine cells but not the parietal or chief cells that are prominent in the adjacent oxyntic mucosa. Cardiac glands are usually branched and connect with relatively short gastric pits. The functional properties of cardiac glands include the secretion of mucus.
2 Oxyntic glands are the most distinctive feature of the human stomach. They occupy the fundus and body of the stomach and contain the oxyntic or parietal cells, which are the sites of acid production. Oxyntic glands also contain chief cells, the site of gastric pepsinogen synthesis. The tubular oxyntic glands are usually relatively straight but sometimes branch; several glands may empty into a single gastric pit. The glands are divided into three regions: (a) the isthmus, containing surface mucous cells and a few scattered parietal cells; (b) the neck, with a heavy concentration of parietal cells and a few neck mucous cells; and (c) the base of the gland, containing chief cells, undifferentiated cells, a few parietal cells, and some mucous neck cells. Endocrine cells are scattered throughout all three regions of oxyntic glands.
The most distinctive cell of the gastric mucosa is the acid-secreting parietal cell. Parietal cells have an unusual ultrastructural specialization in the form of intracellular canaliculi, a network of clefts extending to the basal cytoplasm and often encircling the nucleus, which is continuous with the gland lumen (Fig. 44-6). The surface area provided by the intracellular secretory canaliculi is large and is further magnified by microvilli lining the canaliculi. In parietal cells that are not stimulated to secrete acid, the secretory canaliculi are collapsed and inconspicuous. On stimulation, a severalfold increase in canalicular surface area occurs, the intracellular clefts become prominent, and the communication with the luminal surface is readily identified. These changes create an intracellular space in communication with the gastric lumen into which hydrogen ions are secreted at high concentration.
The cytoplasm of the parietal cell also contains an abundance of large mitochondria. Mitochondria are estimated to occupy 30% to 40% of the cytoplasmic volume of unstimulated parietal cells, reflecting the extremely high oxidative activity of these cells. The oxygen consumption rate of isolated parietal cells is approximately five times higher than that of gastric mucous cells. The cytoplasm also contains a limited amount of rough endoplasmic reticulum, presumed to be the production site of intrinsic factor, which is also secreted by parietal cells.
Figure 44-6. Resting and stimulated parietal cell, emphasizing morphologic transformation with increase in secretory canalicular membrane surface area that occurs with acid secretion.
Figure 44-7. Contrasting morphology of antral gastrin cell (left) with basally oriented secretory granules, and gastric mucous cell (right) with apical mucous granules.
In addition to parietal cells, the oxyntic glands contain the gastric chief cells, which synthesize and secrete pepsinogen. Chief cells are most abundant in the basal region of the oxyntic glands. The cells have a morphology typical of protein-secreting exocrine cells and are similar in ultrastructural appearance to pancreatic acinar cells. Rough endoplasmic reticulum is abundant in the cytoplasm and extends between secretory granules. Zymogen granules containing pepsinogen are most concentrated in the apical cytoplasm. Pepsinogen is released by exocytosis from secretory granules at the apical surface of chief cells.
Antral glands occupy the mucosa of the distal stomach and pyloric channel. Antral glands are relatively straight and often empty through deep gastric pits. Although most cells in the antral glands are mucus secreting, gastrin cells are the distinctive feature of this mucosa. Gastrin cells are pyramid shaped, with a narrow area of luminal contact apically and a broad surface overlying the lamina propria basally (Fig. 44-7). Gastrin cells are identified immunocytochemically by the presence of the peptide. Granules ranging from 150 to 400 nm in diameter are the sites of gastrin storage and are most numerous in the basal cytoplasm. Gastrin is released by exocytotic fusion of the secretory granule with the plasma membrane. In contrast to secretion from chief cells, emptying of gastrin-containing granules occurs at the basal membrane rather than at the apical region of the cell. Gastrin thus released diffuses to and enters submucosal capillaries in close apposition to the lamina propria.
GASTRIC PEPTIDES
The stomach contains a number of biologically active peptides in nerves and mucosal endocrine cells, including gastrin, somatostatin, ghrelin, gastrin-releasing peptide, vasoactive intestinal polypeptide (VIP), substance P, glucagon, and calcitonin gene–related peptide. The peptides with the greatest importance to human disease and clinical surgery are gastrin, somatostatin, and ghrelin.
Gastrin
The synthesis, secretion, and action of gastrin have been extensively studied, and many aspects of the biology of gastrin appear to be shared by other gastrointestinal peptide hormones.1 The gene that encodes for gastrin was isolated using a human DNA library. The human gastrin gene contains three exons; two exons consist of coding sequences. The major active product is encoded by a single exon. In adults, the gastrin gene is expressed primarily in mucosa cells of the gastric antrum, with lower levels of expression in the duodenum, pituitary, and testis. During embryonic development, the gastrin gene is transiently active in pancreatic islets and colonic mucosa.
The human gene encompasses approximately 4,100 base pairs and directs the synthesis of a peptide of 101 amino acids (Fig. 44-8). The resulting peptide, preprogastrin, contains the sequence of gastrin within its amino acid sequence. Preprogastrin consists of a signal peptide of 21 amino acids, an intervening peptide of 37 amino acids, the 34-residue region of the gastrin molecule, and a carboxyl-terminal extension of nine amino acids. Gastrin is derived from its preprohormone by the sequential enzymatic cleavage of the signal peptide, the intervening peptide, and the carboxyl-terminal extension.
The signal peptide region of preprogastrin consists of a series of hydrophobic amino acids that direct the nascent peptide into the endoplasmic reticulum as it is translated from messenger RNA. After directing the preprogastrin molecule into the rough endoplasmic reticulum, the signal peptide is removed. The remaining peptide is termed progastrin. Progastrin is further processed as it traverses the endoplasmic reticulum to mature secretory vesicles. Enzymatic cleavage at a pair of basic amino acid residues proximal to the gastrin 34 (G34) sequence removes the intervening peptide. A similar cleavage removes a six-amino-acid fragment at the carboxyl-terminal end. The peptide that remains has a Gly-Arg-Arg sequence at the carboxyl terminus. Carboxypeptidase cleaves the Arg residues, and the peptide that results is termed glycine-extended gastrin. G34 is formed by cleavage of the Gly-Arg-Arg sequence and amidation of the carboxyl-terminal phenylalanine. Gastrin, like most gastrointestinal peptide hormones, requires terminal amidation for biologic activity. Gastrin 17 (G17), the most abundant form of gastrin in the human antrum, is formed by further processing that removes the first 17 amino acids at the amino terminus of G34. G34 is the predominate molecular form of gastrin in the duodenum.
Figure 44-8. Sequential processing of preprogastrin molecule.
3 The most important stimulant of gastrin release is a meal. Small peptide fragments and amino acids that result from intragastric proteolysis are the most important food components that stimulate gastrin release. The most potent gastrin-releasing activities are demonstrated by the amino acids tryptophan and phenylalanine. Ingested fat and glucose do not cause gastrin release. Gastric distention by a meal activates cholinergic neurons and stimulates gastrin release. As the meal empties and distention diminishes, VIP-containing neurons are activated, which stimulate somatostatin secretion and thus attenuate gastrin secretion.
Postprandial luminal pH also strongly affects gastrin secretion. Gastrin release is inhibited when acidification of an ingested meal causes the intraluminal pH to fall below 3.0. Conversely, maintaining intragastric pH above 3.0 potentiates gastrin secretion after ingestion of protein or amino acids.2 Pernicious anemia and atrophic gastritis, which produce chronic achlorhydria, are associated with fasting hypergastrinemia and an exaggerated gastrin meal response. Release of mucosal somatostatin occurs with gastric acidification and this peptide has been implicated in the inhibition of gastrin release that occurs when luminal pH falls.
The vagus nerve appears to both stimulate and inhibit gastrin release.3 In humans, vagally mediated stimulation of gastrin release can be demonstrated by sham feeding, insulin-induced hypoglycemia, and administration of the vagal stimulant gamma-aminobutyric acid. In contrast to these stimulatory vagal effects, hypergastrinemia, observed after vagotomy, suggests that inhibitory vagal effects on gastrin release may also exist. Cholinergic neurons stimulate gastrin secretion directly by actions on gastrin cells. By decreasing somatostatin secretion, cholinergic neurons also indirectly stimulate gastrin release. Evidence suggests that vagal stimulation of gastrin release is mediated by bombesin or its mammalian equivalent, gastrin-releasing peptide, acting as a neurotransmitter in the gastric wall. Adrenergic stimulation has also been noted to increase gastrin release.
Chronic gastric infection with Helicobacter pylori causes increased acid secretion by altering gastrin release.4,5 H. pylori has been observed to upregulate proinflammatory cytokines, including interleukin (IL)-6, IL-8, and tumor necrosis factor-α (TNF-α). Several inflammatory mediators have been demonstrated to stimulate gastrin release from isolated gastrin cells. The putative gastrin secretagogues include IL-1, IL-8, TNF-α, interferon-gamma, and leukotrienes C4 and D4. The same factors that affect gastrin release also influence gastrin mRNA expression. Food ingestion increases gastrin mRNA abundance, whereas fasting and somatostatin decrease gastrin mRNA production. Chronic achlorhydria, as seen in pernicious anemia, increases gastrin mRNA production.
In addition to stimulating acid secretion from gastric parietal cells (detailed later in this chapter), gastrin has important physiologic actions in the control of gastrointestinal mucosal growth. The acid-secreting oxyntic mucosa is particularly sensitive to the trophic actions of gastrin, but the mucous membranes of the duodenum, colon, and pancreatic parenchyma are also affected. Stimulation of mucosal growth by gastrin is enhanced by the presence of solid food in the diet. The 17- and 34-amino-acid forms of gastrin are equipotent in stimulating mucosal growth. Prolonged stimulation by high levels of gastrin, as seen in the Zollinger–Ellison syndrome, is associated with hypertrophy of the gastric mucosa. Smaller increases in circulating gastrin, such as those that follow vagotomy, do not cause mucosal hypertrophy.
Figure 44-9. Derivation of somatostatin 14 from preprosomatostatin precursor.
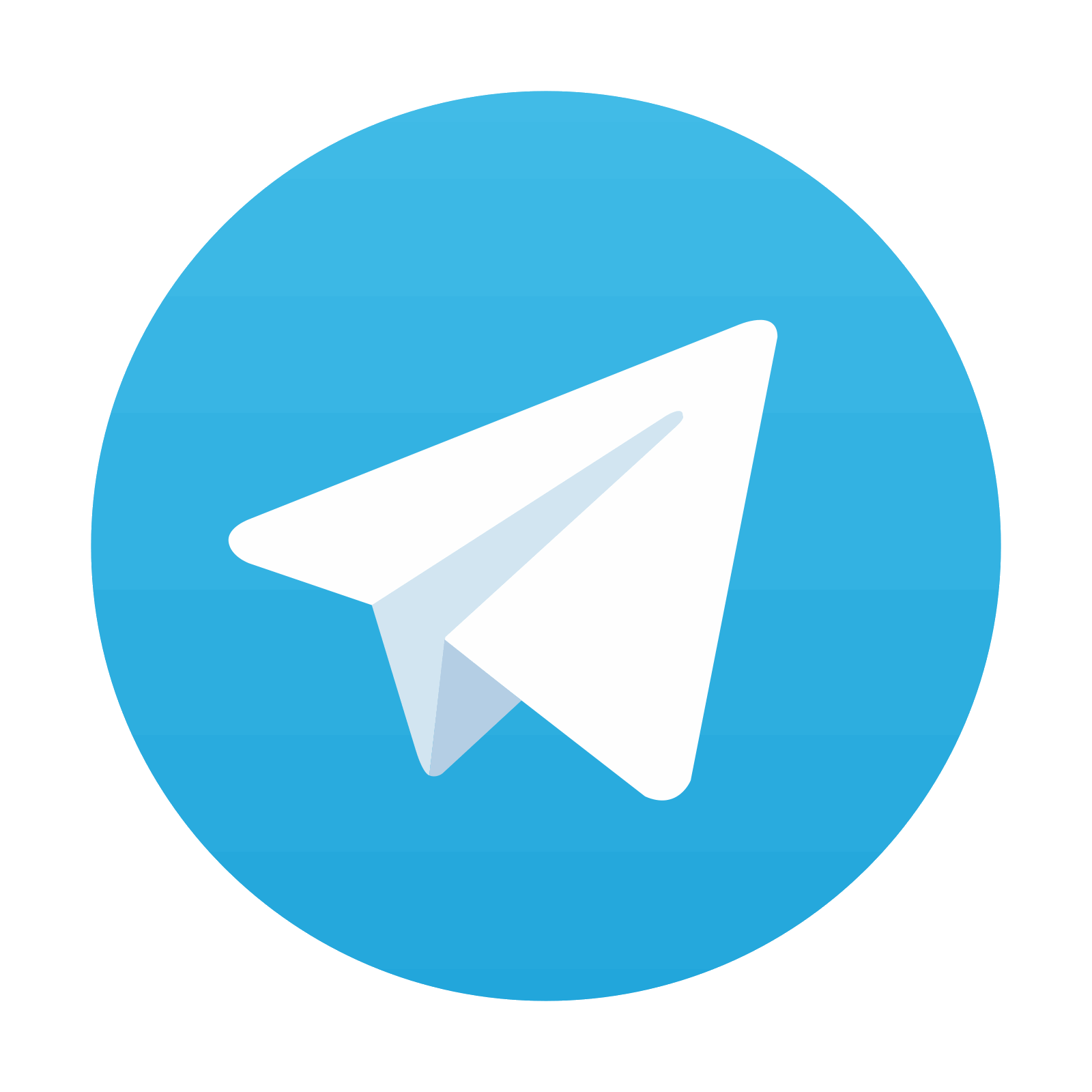
Stay updated, free articles. Join our Telegram channel
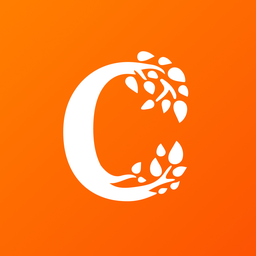
Full access? Get Clinical Tree
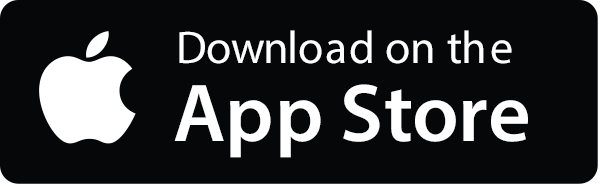
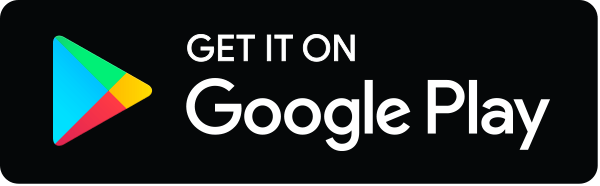