Name
Location
Type
FRAXA
Xq27.3
Rare
FRAXB
Xp22.31
Common
FRAXC
Xq22.1
Common
FRAXD
Xq27.3
Common
FRAXE
Xq28
Rare
FRAXF
Xq28
Rare

Fig. 19.1
Appearance of FRAXA. (a) Conventional stain (Giemsa) and (b) GTG-banded. The arrow indicates the location of the fraX site
FraX is not a chromosome abnormality. It is a chromosomal “marker” that facilitated the diagnosis of FXS until better techniques were developed.
Cytogenetic Expression in Affected Males and Carrier Females
In affected males, fraX expression varied from less than 49% to 50%, with the low-expressing males comprising a minority of the diagnosed cases. However, this group represents the false-negative males diagnosable with molecular techniques. Why fraX does not express in >50% of metaphases is still not known. Cytogenetic testing of carrier (heterozygous) females was even more problematic. Among obligate carriers, only about 50% tested positive, and about one-third of these carriers were clearly affected to some degree. In general, fraX expression was easier to demonstrate (although lower than in males) in affected females than in those with normal intelligence. Guidelines were established for interpretation of these data [14, 15].
Prenatal Diagnosis
Prenatal testing was available on an experimental basis beginning in 1981 using cytogenetic techniques. Testing was done on fetal blood, amniocytes, or chorionic villus cells with varying degrees of success. False-negative males were reported with all three tissue types. In the United States, amniocentesis was the major procedure, while chorionic villus sampling (CVS) was the standard in Europe and Australasia at this time. England had the major experience with fetal blood sampling. Worldwide experience with prenatal diagnosis by cytogenetic analysis or cytogenetic analysis plus DNA polymorphism analysis (see discussion later) exceeded 400 cases. The “state of the art” was summarized at the Fourth International Workshop on Fragile X and X-Linked Mental Retardation. Fortunately, with the identification of the molecular defect in FXS, prenatal diagnosis of the syndrome became much more accurate.
Molecular Aspects of Fragile X Syndrome
Analysis Using Linked Polymorphisms
From the mid-1980s through 1991, molecular (DNA) analysis using linked polymorphisms was used in confirmed fraX families to help with prenatal diagnosis and carrier status. Although the gene for FXS had not been identified, its relative location on a linear map of the distal X long arm was known. Using genes and polymorphisms on both sides of fraX allowed molecular geneticists to track fraX chromosomes through families. The risks of inheriting the fraX chromosome were expressed as probabilities. Success with the method depended on the distance between the tested polymorphism/gene and the FXS gene, the size of the family, and which polymorphism/genes were informative. Regardless of these limitations, the combination of cytogenetic and linkage analysis allowed many families to receive more reliable results than with chromosome analysis alone.
Trinucleotide Repeats: Classification
The early 1990s marked the discovery of a new type of genetic mutation in humans: the trinucleotide or dynamic repeat. The mechanism causing the FXS mutation was first identified in 1991 and revealed that the mutation results from the expansion of a trinucleotide repeat located in or near an expressed sequence [16–19]. For the fragile X syndrome, the trinucleotide repeat is cytosine-guanine-guanine or CGG. This revelation was soon followed by the discovery that a similar mechanism causes myotonic dystrophy (DM) and spinocerebellar ataxia type 1 (SCA1). To date, more than 30 human diseases known to be associated with the expansion of a trinucleotide repeat [20–22].
Trinucleotide repeat disorders can be categorized in one of two ways: (1) according to the specific trinucleotide sequence or (2) according to the location of the expansion in relation to the coding sequence. Here, we have chosen to describe trinucleotide repeat disorders based on the position of the expansion in relation to the coding sequence. The repeats may be located in the 5′ untranslated region, in an intron, in an exon, or in the 3′ untranslated region. The list of disorders continues to grow. One characteristic of these disorders, that each generation shows an earlier age of onset and increasing severity, is known as anticipation. All the disorders are either X-linked or autosomal dominant except Friedreich ataxia, which is autosomal recessive [22].
The CGG trinucleotide repeats are located at folate-sensitive fragile sites, and their characteristics are summarized in Table 19.2 [23, 24]. Based on the trinucleotide repeat size in FRAXA, an individual’s status can be classified as normal (5–44 CGG repeats), indeterminate or gray zone (45–54 CGG repeats), premutation (55–200 CGG repeats), or full mutation (>200 CGG repeats) [25]. An individual with a normal repeat size is characterized by stability of the repeat length and normal intelligence, while an individual with a premutation repeat size shows instability of the repeat length from generation to generation but normal intelligence. In contrast, full mutation individuals have massive repeat sizes differing in lengths (“mosaic”) in a pattern that is often conserved across tissues, resulting in FXS.
Table 19.2
Classification of trinucleotide repeat diseases
Class | n | Repeat | Position of Repeat | Examples |
---|---|---|---|---|
1 | 3 | CGG | 5′ Untranslated region | FXS |
FRAXE syndrome | ||||
1 | CAG | Spinocerebellar ataxia type 12 (SCA12) | ||
2 | 2 | CTG | 3′ Untranslated region | Myotonic dystrophy (DM) |
3 | 8 | CAG | Inside coding region | Huntington’s disease (HD) |
Spinocerebellar ataxia type 1 (SCA1) | ||||
Kennedy’s disease | ||||
4 | 1 | GAA | In first intron | Friedreich Ataxia |
Instability of the CGG Repeat
Through observational studies of families with FXS, several factors involved in CGG repeat instability have been proposed, including the sex of the transmitting parent, the size and structure of the CGG repeat, and other yet-to-be-identified factors. With the resolution of the Sherman paradox, it is now known that a premutation-sized repeat has the propensity to expand when passed through a female germ line, and the size of the resulting expansion is positively correlated with the maternal repeat size [26–30]. In contrast, when passed through a male germ line, the premutation does not dramatically change in repeat size and often remains the same or even contracts [29–31].
In addition to the sex of the transmitting parent, the size and structure of the CGG repeat play a role in instability. Sequencing of the CGG repeat revealed that the repeat is not pure and is interspersed with one to three AGGs (adenine-guanine-guanine sequences) every 9–10 CGGs in the general population. Among families with FXS, premutation-sized repeats usually have one AGG at the most proximal end of the repeat or none at all [32–34]. Transmission studies of families with premutation- or intermediate-sized repeats demonstrate that these are unstable if >34 repeats at the 3′ end of the repeat structure are uninterrupted by an AGG [29, 32, 34]. To date, all known expansions have occurred at the 3′ end of the repeat. This polarity of expansion further demonstrates the importance of the 3′ end of the repeat in the expansion process. While the role of the AGG interruption has only been minimally defined by experimental studies, these observational and population studies suggest that the AGG sequence acts as an anchor during DNA replication to prevent expansions or deletions that are the result of slips or misalignments of the repeat sequence during replication [35–38]. Despite the identification of these factors, the exact mechanism of the formation of the repeat expansion has not been completely elucidated.
Many models have been proposed to explain the expansion of trinucleotide repeats. One of the first proposed mechanisms involved in repeat instability at the molecular level was slippage of the replication fork during DNA synthesis. Unpaired bases form loops, which result in expansions or contractions in the next round of replication, depending on whether the looped repeats are located in the newly synthesized or template strand [39]. However, slippage alone cannot explain all aspects of repeat expansions, especially large expansions and contractions. It is clear that other yet-to-be-identified factors are involved in the expansion process. Experimental support has come from studying a yeast model deficient in rad27 [40]. The Rad27 protein is involved in removing DNA loops, such as those arising during displacement synthesis of the Okazaki fragments. Propagation of a CGG repeat in rad27 null yeast results in a highly significant increase of repeat expansions [40]. The human homolog of this gene is FEN1.
Recognition of the unusual structural properties of trinucleotide repeats yielded new insights. Disease-causing repeats are almost exclusively formed by (CNG)n triplets. Single-stranded (CNG)n can form hairpin-like structures that can include both Watson-Crick and mismatched base pairs. Due to their different sequences, the leading and lagging strands have different tendencies to form hairpins. The secondary structures are likely to affect recognition and subsequent repair or recombination of the structure [41, 42]. Unusual DNA structures may stall DNA polymerase, leading to instability. A complex model based on replication fork stalling and restarting has been described in detail [43].
Unlike other trinucleotide repeat disorders, there is absence of repeat instability in somatic cells in FXS. Methylation may stabilize the CGG repeats in full mutations (see section, “Epigenetic Changes in the FMR1 Gene”). Repeat expansion from pre- to full mutation occurs exclusively in females due to sex-specific factors. It has long been known that sperm from full-mutation male patients possess only premutation alleles [44]. This must result from reduction of repeat instability occurring during a limited time in early development. Both prezygotic and postzygotic models, directly after separation of the germ cells, have been proposed [45]. Material is not available from premutation females to support either hypothesis.
The Fragile X Gene and Its Product: FMR1 and FMRP
In 1991, the responsible gene was identified by positional cloning and named the fragile X mental retardation-1 (FMR1) gene [17–19]. FMR1 encompasses 38 kb of Xq27.3 and consists of 17 exons [46]. The polymorphic CGG repeat exists in the 5′ untranslated region (UTR) of FMR1. Among the general population, the CGG repeat ranges from 6 to 55 repeats and usually the size does not change in size when passed from parent to offspring [26]. The most common forms of the repeat sizes found in human populations studied are 21 and 28–30 CGG repeats [47–50]. Although the CGG repeat has no known function, it is found in all species of mammals investigated [51, 52].
The common CGG-repeat sizes have not proven to be associated with a disease phenotype; however, the consequence of an expanded CGG repeat (>200 repeats) in FMR1 is the fragile X syndrome. The hyperexpanded CGG repeat signals the hypermethylation and deacetylation of the FMR1 promoter, the CGG repeat, and a nearby CpG island, which transcriptionally silences the gene [17, 53–56]. Recent in vitro experiments demonstrated that it is methylation and chromatic modification triggered by the expansion that are responsible for the transcriptional silencing of FMR1 rather than the CGG repeat expansion itself [57, 58] (see also section, “Epigenetic Changes in the FMR1 Gene”).
Because FXS is essentially caused by the loss of the FMR1 gene product, there is much interest in gathering information on the normal expression patterns of the gene and its product’s function for the development of interventions or therapies. The FMR1 transcript is approximately 4.4 kb in size and is alternatively spliced at the 3′ end, giving rise to various isoforms [46, 59]. Expression studies in human and mouse tissues demonstrated that FMR1 is widely expressed, with the highest levels localized to the brain, testes, ovaries, esophageal epithelium, thymus, spleen, and eye [60–62]. High expression of FMR1 in regions of the brain such as the neurons of the hippocampus and the granular layer of the cerebellum is consistent with the mental retardation phenotype typical of FXS [63, 64] (see also section, “Clinical Aspects of Fragile X Syndrome”). Identification of other mutations (e.g., deletions and point mutations in patients with FXS) has confirmed that FMR1 is the only gene involved in the pathogenesis of the disorder and that the loss of the FMR1 product causes the syndrome.
A search for genes similar to FMR1 within the human genome found two identified autosomal homologs, fragile X-related (FXR) genes 1 and 2, located at 3q28 and 17p13.1, respectively [65, 66]. Analysis of mouse and human genomic sequences demonstrates similarities in gene structure among FMR1, FXR1, and FXR2, suggesting that the three genes have an ancestral gene in common [67]. The functions of FXR1 and FXR2 are presently unclear; neither gene has been shown to be associated with human disease. Many investigators have postulated that, because of their similarity to FMR1, the FXR genes are somewhat redundant, but although there are similarities, significant differences have been noted [68]. Furthermore, FXR1 and FXR2 are not overexpressed in cells from persons with FXS, suggesting that neither gene product compensates for the loss of the FMR1 gene product [69, 70].
The full-length protein product of FMR1 is 69 kilodaltons in size and is known as the fragile X mental retardation protein, or FMRP [71]. At the protein level, FMRP is highly conserved across humans, mice, the African clawed frog (Xenopus laevis), and chickens [18, 59, 72, 73]. Although not as highly conserved as among vertebrates, a homolog for the FMR1 coding sequence has also been identified in Drosophila melanogaster [74].
Much has been accomplished in elucidating the function of FMRP and how its absence leads to the development of the FXS phenotype. Several properties of FMRP were the first clues to its function. First, FMRP contains two ribonucleoprotein K homology domains (KH domains) and clusters of arginine and glycine residues (RGG boxes), features typical of RNA-binding proteins [71, 75]. Second, FMRP contains both a nuclear localization signal (NLS) and a nuclear export signal (NES) [76]. Two coiled coils and a G-quartet-binding structure have been identified (Fig. 19.2) [77]. FMRP is primarily a cytosolic protein, but its presence in the nucleus has been reported by nuclear staining experiments [63, 78]. Furthermore, FMRP has been detected in the nuclear pore [79]. Taken together, current evidence suggests that FMRP shuttles between the nucleus and the cytoplasm affecting protein synthesis in dendrites (dendritic spines) and synapses [76, 80–82].


Fig. 19.2
The FMR1 gene-coding exons (numbered boxes) and protein domains. NLS nuclear localization signal, KH1/KH2 RNA-binding domains, NES nuclear export signal, RGG RGG box RNA binding. The triangle indicates the untranslated CGG repeat alleles, <45 = normal range, 45–54 = gray zone, 55–200 = fragile X premutation, >200 = fragile X full mutation (Modified from Schneider et al. [77]; reprinted with permission)
Dendritic spines are small membranous extensions on neuronal dendrites [83]. They serve as synaptic storage sites, support the electric signal transmission, and increase the number of possible contacts between neurons [84].
On their surface, the dendritic spines express glutamate receptors (GluR) of two types: the ionotropic receptors alpha-amino-3-hydroxy-t-methyl-4-isoxazolepropionic acid (AMPA) and N-methyl-d aspartic acid (NMDA), and the metabotropic receptors (mGluRs). A broad variety of proteins mediate the signaling from the GluRs [85].
Cognitive function, motivation, learning, and memory are based on spine plasticity. After the formation of numerous dendritic spines during fetal cortical neurogenesis, the spines need to mature or be pruned; immature spines show a significant impairment in signal transduction. Abnormalities in spine formation have been observed in FXS, which can be directly correlated to the cognitive impairment. It has been postulated that the loss of FMRP in FXS leads to an excessive expression of mRNA near synapses, making it impossible to regulate protein synthesis adequately, thus increasing long-term depression due to receptor loss. FMRP is considered to be a repressor of specific mRNA translation and numerous proteins are upregulated, particularly in the hippocampus, when FMRP is absent [86].
FMRP colocalizes primarily with polyribosomes and ribosomes at/in the endoplasmic reticulum membrane and appears to play an important role in the regulation of translation of specific target mRNAs. A subset of mRNAs containing a G-quartet (a nucleic acid structure in which four guanine residues are arranged in a planar configuration) has been identified that are potential targets for FMRP, including important neuronal proteins like microtubule-associated protein 1B (MAP1B) and semaphorin 3F [87–89]. FMRP also can bind to mRNAs that do not contain a G-quartet.
In addition, experimental evidence suggests that FMRP is involved in suppression of translational activities. FMRP forms complexes with messenger ribonuclear particles (mRNP) and is associated with translating ribosomes [76, 90, 91]. Because RNPs are formed in the nucleus, this observation further supports the hypothesis that FMRP shuttles between the nucleus and the cytoplasm. Recent experiments suggest that FMRP may play a role in regulation of translation for certain messages. Laggerbauer et al. demonstrated that FMRP suppresses translation by preventing the assembly of the 80S subunit of the ribosome on the target RNAs [92]. New evidence suggests that translational control may be mediated through the RNA interference (RNAi) and/or microRNA (miRNA) pathways [93, 94].
The two major activities identified for FMRP, cytoplasm-nucleus shuttling and translational regulation, imply that FMRP is a facilitator for the expression and localization of several messages and proteins. The search for FMRP’s partners has identified at least seven such proteins, one of which includes FMRP itself [68]. In contrast, very few specific mRNAs that bind FMRP have been identified.
FMRP was shown to bind its own mRNA and also approximately 4% of fetal brain mRNAs [71]. Nearly a decade would pass before the identity of the specific mRNAs (other than the FMR1 transcript) binding to FMRP would be identified [91–93]. These mRNAs contain a G-quartet structure that facilitates binding to FMRP. FMRP can be phosphorylated, a mechanism that possibly affects the binding of specific mRNAs [94].
The ability of FMRP to bind RNA and suppress translation has definite clinical relevance. As an RNA-binding protein, FMRP is found to form a messenger ribonucleoprotein (mRNP) complex that associates with translating polyribosomes [95]. FMRP is also known to be involved in translational control and could suppress translation both in vitro and in vivo [86]. In 1987, Davis et al. demonstrated that the mRNAs were transported into dendrites of cultured hippocampal neurons [96]. Since then, a large number of dendritic localized mRNAs have been identified, and it is suggested that the translation of those mRNAs can be regulated in a spatially restricted manner in response to stimulation [97]. At the cellular level, abnormal dendritic spines are found in the brains of both human patients with FXS and Fmr1 knockout (KO) mice, implying that synaptic plasticity is affected in the absence of FMRP. Based on these observations, it has been proposed that FMRP is involved in synaptic plasticity via regulation of mRNA transport and local protein synthesis of specific mRNAs at synapses. Transport and regulated translation of mRNAs in dendrites are important for neuronal function, including modulation of synaptic plasticity. This is essential in memory consolidation and learning [84, 98–103]. Altered spine morphology (long and thin dendritic spines) has been observed in postmortem brains of fragile X patients and in Fmr1 KO mice [84, 98–102]. The presence of the protein machinery near synaptic connections allows neurons to rapidly respond to signals at particular synapses through local translation of specific mRNAs in the vicinity of the synapse, and FMRP plays a crucial role. The response is mediated through the action of mGluR activation [77, 104, 105].
It has been proposed that FMRP located at the synapse represses translation of mRNAs encoding proteins that regulate endocytic events involving the AMPA receptor. Upon synaptic stimulation, FMRP may dissociate from these mRNA targets to allow translation and facilitation of AMPA receptor internalization. The model predicts that in the absence of FMRP, the upregulated translation of a subset of mRNAs would result in the perturbation of AMPA receptor internalization dynamics (Fig.19.3) (see Oostra and Willemsen [44] for further discussion).


Fig. 19.3
The CGG repeat in the FMR1 gene. Schematic representation of normal, PM (premutation), and FM (full mutation) alleles of the FMR1 gene and the effect of the expansion on transcription and translation. Methylation due to extensive elongation of the CGG repeat in the 5′-UTR of the FMR1 gene is depicted as a lock (Modified from Oostra and Willemsen [45]; reprinted with permission)
Protein kinases are crucial for the regulation of neuronal development and synaptic transmission upon response to extracellular or intracellular signals. The mGluR theory is in line with the translation control pathways within the dendritic spines; a simplified version is depicted in Fig. 19.4 [44]. Strong evidence supports the postsynaptic FMRP signaling model. Data suggest that dephosphorylation of FMRP may regulate FMRP and that the release of FMRP-induced translational suppression may involve a dephosphorylation signal. Rapid dephosphorylation of FMRP allows target mRNAs to be translated, whereas rephosphorylation represses translation. Several proteins involved in this process have been identified [106, 107].


Fig. 19.4
The mGluR theory: in the absence of FMRP, as in fragile X syndrome, the balance between FMRP and Gp1 mGluRs is lost, and unchecked protein synthesis at the synapse leads to the characteristic features of the disease (Reprinted with permission from Willemsen R, Levenga J, Oostra BA. CGG repeat in the FMR1 gene: size matters. Clin Genet. 2011;80(3):214–5)
Further research is needed to characterize the cascade of signaling upon mGluR activation and the mechanism whereby FMRP phosphorylation regulates translation of target mRNAs.
Epigenetic Changes in the FMR1 Gene
Methylation of the CGG repeat, which occurs in the promoter region of the FMR1 gene, takes place early in embryonic development and is a dynamic process. In early germ cells from female full-mutation fetuses, the FMR1 repeat is fully expanded and unmethylated [108]. In chorionic villus samples from full-mutation fetuses, the expanded repeat is methylated to an increasing degree as development progresses [109]. Almost all FXS patients carry a fully methylated full expansion.
FMR1-Associated Disorders
Allelic Forms of the FMR1 Gene
There are four allelic forms of the gene: normal, intermediate, premutation, and affected. The associated numbers of CGGs for each are defined here; however, the cutoffs for each allelic type have evolved over time and may change with increased empirical data and research.
Normal Alleles: Normal alleles have a range of 5–44 repeats. The most common repeat lengths are 29 and 30 CGG repeats.
Intermediate (Gray Zone, Inconclusive, Borderline): The range from 45 to 54 repeats is intermediate. Alleles in this range can be considered normal in the sense that such alleles have not been observed to expand to a full mutation in a single generation. Moreover, there is no observed increased risk for the specific premutation-associated disorders, although data are limited.
Premutation: Premutation alleles range from 55 to ∼200 repeats. These alleles are long repeat tracks that are unstably transmitted from female parent to child. Premutations are not associated with somatic variation, are not hyper-methylated, and are not associated with classic features of fragile X syndrome. Women with alleles in this range are at risk to have affected children, although all known mothers of affected children have alleles of 59 repeats or higher [112]. Female members of families with CGG repeats in this range benefit from genetic counseling and prenatal diagnosis.
Full Mutations: Full mutations associated with the fragile X phenotype exhibit more than 200 CGG repeats and typically several hundred to several thousand repeats. There is usually broad somatic variation within each patient. Hypermethylation is typically present on most or all copies.
Clinical Aspects of Fragile X Syndrome
Several disorders are now associated with mutations in the FMR1 gene and are reviewed as follows.
Full-Mutation Phenotypes
Physical Phenotype
In males, the classic features of FXS are X-linked mental retardation, macroorchidism, and minor dysmorphic facial features including a long, oblong face with a large mandible and large and/or prominent ears. Pectus excavatum, mitral valve prolapse, and strabismus have also been described in males with FXS. At least 80% of affected males have one or more of these features, but expression varies with age. Other frequent features are a high-arched palate, hyperextensible finger joints, velvet-like skin, and flat feet. Females with a full mutation may express these same features of FXS, depending upon X-inactivation status [22].
Behavioral Phenotype
The behavior of males with the FXS can be quite variable. They show distinct behavioral features in the areas of attention, hyperarousal, social function, anxiety, and aggression [113, 114]. Additionally, they are often diagnosed with autism because they exhibit poor eye contact, hand flapping, and social deficits that are the most prominent features of autism. They also exhibit various degrees of speech delay. Other complicating features can include irritability, hypotonia, and perseveration in speech and behavior. Social anxiety and avoidance are prominent features of FXS in both sexes.
Hagerman reviewed in detail the physical and behavioral phenotype of FXS [113]. The variability of expression makes clinical diagnosis difficult. Therefore, FXS should be considered in the differential diagnosis of all mentally retarded individuals.
Cognitive Phenotype
In males, preliminary evidence suggests that there are specific deficits in arithmetic, visual motor, and spatial skills; short-term auditory, visual, and working memory; executive function; visuospatial-processing abilities; processing of sequential information; and sustained attention. Approximately 85% of males and 25–30% of females with the full mutation have an intelligence quotient (IQ) less than 70. The severity of intellectual impairment is related to FMRP deficiency [77, 114, 115]. Some individuals with only a mild decrease of FMRP may present with a normal or borderline IQ with or without learning disabilities (LD). LD with a normal or borderline IQ is a typical presentation in females with FXS. IQ decreases with age, although the reason for this longitudinal decline is unclear [116]. Adult males with FXS function within the moderate to severely retarded range. IQ is not correlated with the size of the CGG repeat. However, it does appear to be correlated with the mosaic status of the male. Affected males with both somatic full mutation and premutation size repeats or those who are methylation mosaics have higher IQs than the affected males who are nonmosaic or fully methylated. On occasion, such males will test in the normal/low normal range [117].
In females, with FXS cognitive studies indicate specific weaknesses in arithmetic as well as short-term auditory memory and visual-spatial tasks. They also have significant deficits in executive function. Full-mutation females have mean IQs in the low-average range (74–91), and, as in males, the IQ is not correlated with CGG-repeat size. Most studies have found a relationship between IQ and X-inactivation ratios.
Aging in FXS
There is an ever-increasing group of patients with fragile X syndrome. Until recently, there were no studies on the behavior and cognitive problems in aging for those with FXS. Utari et al. studied a group of individuals (44 males and 18 females) with the syndrome who were over 40 years of age [118]. The most frequent difficulties faced by these patients were neurological problems (38.7%), gastrointestinal problems (30.6%), obesity (29.8%), and heart problems (24.2%), which include mitral valve prolapse (MVP), cardiac conduction abnormalities, heart attack, and heart rhythm disorder. Males had a significantly higher percentage of neurologic problems compared with females. However, only movement disorders, including Parkinson’s disease, were significantly different in prevalence between males and females; some of these may be the result of long-term treatment with antipsychotics. An increase in seizures was observed in the older patients as well. It is not known if this represents a second peak in seizure onset or if seizures are a complication of aging.
Other Clinical Aspects
Premutation Carrier Phenotypes
Unlike the full mutation, the existence of a phenotypic consequence of the premutation in males was controversial for some time. However, a specific phenotype has been identified and is referred to as fragile X tremor ataxia syndrome (FXTAS) [121]. Approximately 40% of older males with the premutation will eventually develop FXTAS. The features of this disorder include an intention tremor, ataxia, Parkinsonism, neuropathy, cognitive deficits (particularly executive function deficits with eventual cognitive decline to dementia in some), and autonomic dysfunction including hypertension, impotence, and eventual bladder and bowel incontinence [118, 122–126]. Although some patients with FXTAS have a rapid decline over 5–6 years, others are stable for a decade or two. More rapid decline typically occurs when the features of FXTAS are combined with another disorder. FXTAS occurs in approximately 8% of female carriers [127].
The neuroanatomical hallmark of FXTAS is intranuclear eosinophilic inclusions in neurons and astrocytes throughout the brain, with highest numbers in the hippocampus and limbic system [128]. These inclusions have also been found in Leydig and myotubular cells of the testicles and in peripheral nerve ganglia throughout the body [126]. They contain the excess mRNA and also a number of proteins including lamin A/C and MbP that are dysregulated by the elevated mRNA [129]. The inclusions are probably not pathognomonic; they are a marker for RNA toxicity.
While cognitive or behavioral deficits have not been definitively attributed to the premutation in males, a molecular phenotype related to this repeat size range has emerged. Early on, investigators examined levels of FMR1 mRNA and FMRP from the lymphocytes of carriers of premutation alleles and found that the levels were not significantly different compared with controls [55, 63]. Recent changes in technology, however, have made measurements of FMR1 mRNA more sensitive and accurate [130, 131]. Using this technology, Tassone et al. reexamined the levels of FMR1 mRNA and FMRP in premutation male carriers and found that carriers with 100–200 CGG repeats had a fivefold increase in FMR1 mRNA levels while carriers with 55–100 repeats had a twofold increase compared with controls [132, 133]. Moreover, these high-end premutation carriers (100–200 repeats) had reduced levels of FMRP compared with controls [132]. Additional experiments suggest that the elevated level of FMR1 mRNA is correlated with CGG-repeat size and is not simply a response to decreased levels of FMRP [133, 134].
An RNA gain of function mechanism has been suggested for FXTAS based on the aforementioned observation of elevated levels of CGG containing FMR1 mRNA, along with either no detectable change in FMRP or slightly reduced FMRP levels, observed in peripheral blood leukocytes and brain regions [132, 134–138] of premutation carriers.
Many conflicting reports exist in the literature concerning cognitive, behavioral, and physical phenotypes among female premutation carriers; these reports have been reviewed [139, 140]. For reports on cognitive ability, studies of varying designs have shown that the prevalence of mental retardation, the range of cognitive ability, and the range of IQ scores among adult female premutation carriers did not differ compared with control groups [141, 142]. However, at least two studies have suggested differences among female carriers compared with controls in specific subsets of IQ scores. In terms of a behavioral phenotype related to the premutation, several studies suggest a difference based on specific behavioral or psychological measures among women with premutations compared with controls [123, 143]. However, many of these suggested differences were not replicated in other studies. Lastly, for physical or anthropometric measures, two studies suggest that female premutation carriers do not have the same facial dysmorphic features typically observed in patients with the full mutation, while two studies suggest otherwise [138, 139].
While the existence of a cognitive, behavioral, or physical phenotype among premutation females remains controversial, one consequence is consistently associated with the premutation: Fragile X primary ovarian insufficiency (FXPOI), referred to as premature ovarian insufficiency (POI) in older literature. FXPOI is defined as the cessation of menses before the age of 40 years. In contrast, the mean age of menopause in the general population is 51 years. The first reports of female carriers of the fragile X mutation having FXPOI were anecdotally noted at the first International Fragile X Conference (1987) in Denver, Colorado [144–149]. Schwartz et al. were the first to report an association between the fragile X premutation and POI in a multicenter study [145]. The relationship between the fragile X premutation and POI was eventually confirmed by a large, multicenter study, which demonstrated that 16% of premutation carriers experienced POI, while only 0.4% of noncarriers and none of the full-mutation carriers experienced POI [146]. Results from this collaborative effort conclusively demonstrated that the premutation form of the CGG repeat, not the full mutation, is associated with POI. Also, these data, combined with additional reports from other sites, suggest that the rate of POI among premutation carriers is 21% (95% confidence interval: 15–27%) [147]. Overall, approximately 14% of idiopathic familial POI and 2% of sporadic POI in the general population can be attributed to the fragile X premutation allele [147].
The cause of POI among premutation carriers is related to excess mRNA produced by the cells. Many models have been proposed to explain the role of the premutation allele (as opposed to the full-mutation allele) in the development of POI among many (but not all) premutation carriers, but recent studies have yielded few clues to lend support to any one model. Regardless of the cause, the occurrence of POI is one of the factors that can limit the usefulness of preimplantation genetic testing (PGT) as a reproductive option for carrier females [150–152]. In fact, recent hormonal studies suggest that female premutation carriers may unknowingly be experiencing ovarian dysfunction at an early age and may be facing a poorer prognosis for future pregnancy much earlier than expected [153]. The objective of PGD for FXS is to utilize only those embryos that receive the normal X chromosome from the mother. Donor egg, where available, is another reproductive option that allows carrier females, even those with POI, to have unaffected children [154].
It is important to recognize that there are other medical and psychiatric problems that can occur in some carriers, and these are not necessarily part of FXTAS or FXPOI but may nevertheless be related to mRNA toxicity. Neuropathy is relatively common in older carriers and can occur without other symptoms of FXTAS. Hypertension is seen in the majority of older carriers and may be secondary to the autonomic dysfunction related to RNA toxicity [127]. Autoimmune problems may be more frequent in female premutation carriers [127].
Psychopathology that is more common in those with the premutation includes anxiety, depression, and obsessive compulsive behavior; these problems are clinically significant for 25–40% of carriers [155, 156]. In addition, there is newer evidence that the premutation has a neurodevelopmental component in some children, especially boys, causing a higher incidence of ADHD, shyness, and social deficits including autism spectrum disorder [123, 157, 158]. Further studies of premutation carriers identified during newborn screening may further delineate the percentage of carriers with these problems.
Intermediate Carriers
Intermediate alleles, also known as “gray-zone” alleles, range from 45 to 54 CGG repeats and are classified differently than premutation or common alleles in that they may or may not be transmitted unstably from parent to offspring [29]. Intermediate alleles, like premutation alleles, do not cause hypermethylation of the CpG island near FMR1 and are not thought to affect cognitive or behavioral development. However, a recent study from Wessex, United Kingdom, found that boys placed in special education had a higher frequency of alleles in the intermediate and premutation range compared with controls [159]. The results from these data suggested, for the first time, that large CGG repeats smaller than premutations were somehow responsible for the child’s placement in special education [49, 159]. Although an excess of intermediate and premutation alleles has not been observed in other special education populations, new cognitive and molecular data warrant further research to identify and define a phenotypic consequence of intermediate-repeat alleles of FMR1, if one exists [132, 160, 161].
Timing of the Premutation Expansion
One of the yet unsolved questions is when in development the expansion from premutation to full mutation occurs. Expansion could occur during oögenesis (meiotic) or after fertilization (mitotic). Reyniers et al. showed that full-mutation or mosaic full/premutation males produce only premutation sperm and therefore premutation daughters, since repeat expansion occurs only in females [26, 162]. Testicular selection against full-mutation sperm is unlikely, since male Fmr1 knockout mice show fertility [163]. These data support a model of expansion only in somatic cells and protection of the premutation in the germ line cells. However, Malter et al. showed that, in full mutation fetuses, only full-mutation alleles (in the unmethylated state) were found in oöcytes from intact ovaries or in immature testes from 13-week fetuses, but that both full and premutation alleles were found in the germ cells of a 17-week male fetus [164]. They hypothesize that the full mutation contracts in the fetal testes, with subsequent selection for the premutation sperm. In females, the expansion could occur during maternal oögenesis or very early in embryogenesis prior to general methylation. The answer requires analysis of oöcytes from premutation females.
Current Genetic Aspects of Fragile X Syndrome
Epidemiology
Crawford et al. provided an extensive review of the literature and indicated a prevalence of FXS ranging from 1 in 3,717 to 1 in 8,198 in Caucasian males in the general population [165]. The female prevalence rate is presumed to be approximately one-half of the male rate. In another study carried out over 4 years in metropolitan Atlanta, Crawford et al. determined the prevalence of the FXS to be 1 in 2,545 African-American males and 1 in 3,717 Caucasian males [166]. However, the prevalence estimate for Caucasian males, determined from this and from other studies, fell within the 95% confidence interval for African-American males. The prevalence of the fragile X mutation in an Afro-Caribbean population in the French West Indies was similar (1 in 2,539) to that in the African-American population in Atlanta [167]. Falik et al. have suggested that the Tunisian Jewish population is the only other ethnic group to have a higher prevalence of FXS than the Caucasian population [168]. However, these studies were not supported by the data of Tolodano-Alheder et al. [169]. Further studies are required to determine if the frequency of FXS differs in ethnic populations. A recent systematic review of population screening for fragile X syndrome summarized the data and suggested that if population screening is to be instituted more psychosocial support will be required [170].
The premutation is common in the general population with a prevalence of 1 in 130–260 females and 1 in 250–810 males [171]. There is variability in the prevalence figures depending on where the study was done and the ethnic or racial background of the patients. The study recently reported by Cronister et al. in 2008 has shown that the premutation is less common in those of Chinese background and more common in the Middle East, particularly in Israel, as reported by others [169, 172].
The premutation form of the CGG repeat is the precursor to the full mutation in that the repeat is very unstable when transmitted from parent to offspring, eventually expanding to the full-mutation form when passed through a female germ line [27]. Using this definition, premutations can range from 50 to <200 repeats. The absolute lower boundary of the premutation repeat size that is at risk for expanding to the full mutation in a single generation is still under debate [25, 173]. Studies of premutations among families with a member reported affected by FXS suggest that the smallest premutation to expand to the full mutation in a single generation is 59 repeats [173]. However, small premutation alleles (∼50–65 repeats) ascertained from the general population have proven to be more stable than those ascertained from families with FXS [173]. Given the uncertainty in the lower boundary of the premutation, the prevalence of the premutation varies from study to study, depending on the ranges of CGG repeats that are considered premutations.
Molecular Rules of Inheritance
DNA analysis of the FMR1 allele can detect all stages of the trinucleotide repeat expansion. Reduced penetrance, the Sherman paradox, and other unusual characteristics of FXS were explained by the silent premutation state. The rules of inheritance, as currently understood, include the following [174]:
1.
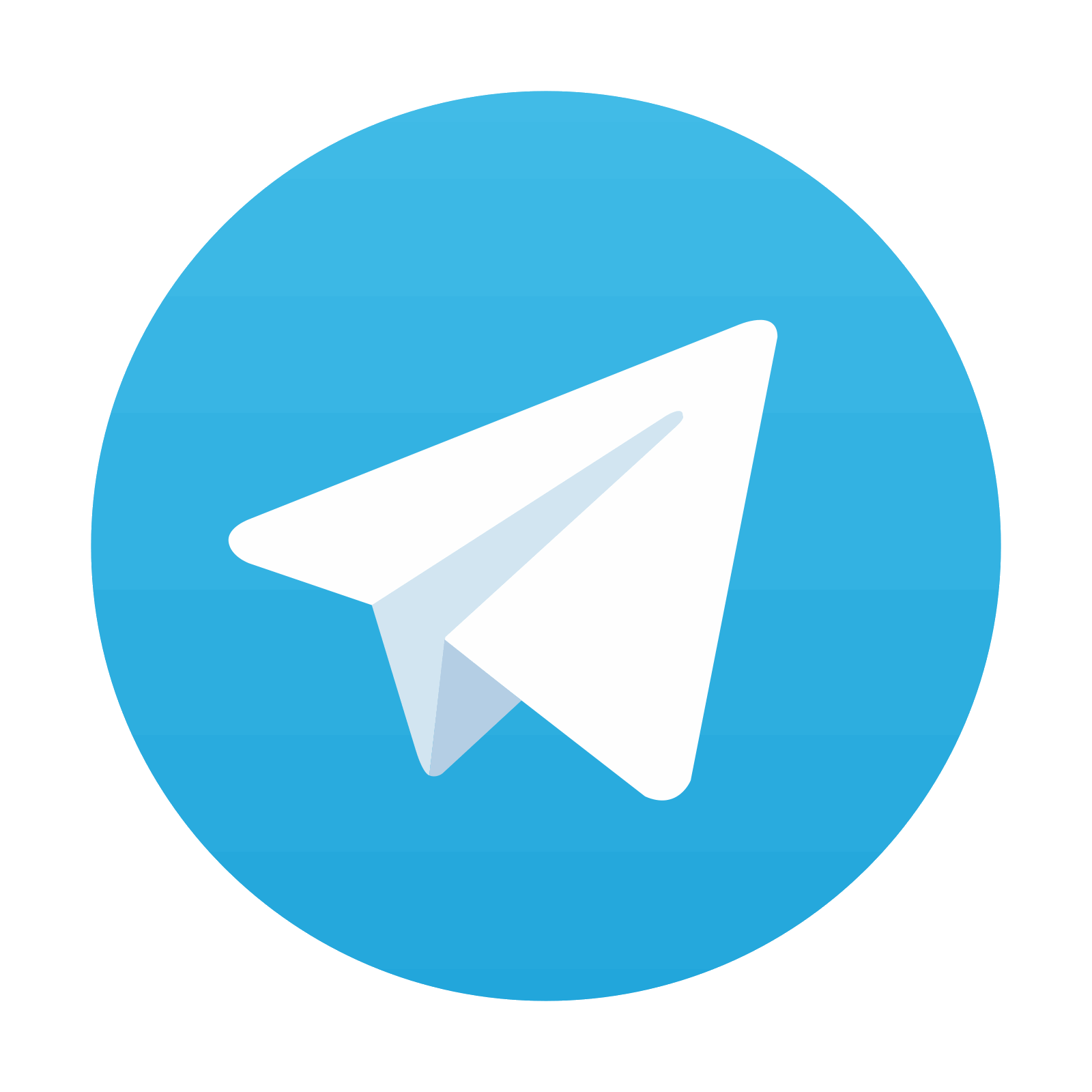
Every affected individual has a carrier mother with an observable expansion. No new mutation has gone directly from normal to full. Full-mutation males do not pass a full mutation to their daughters.
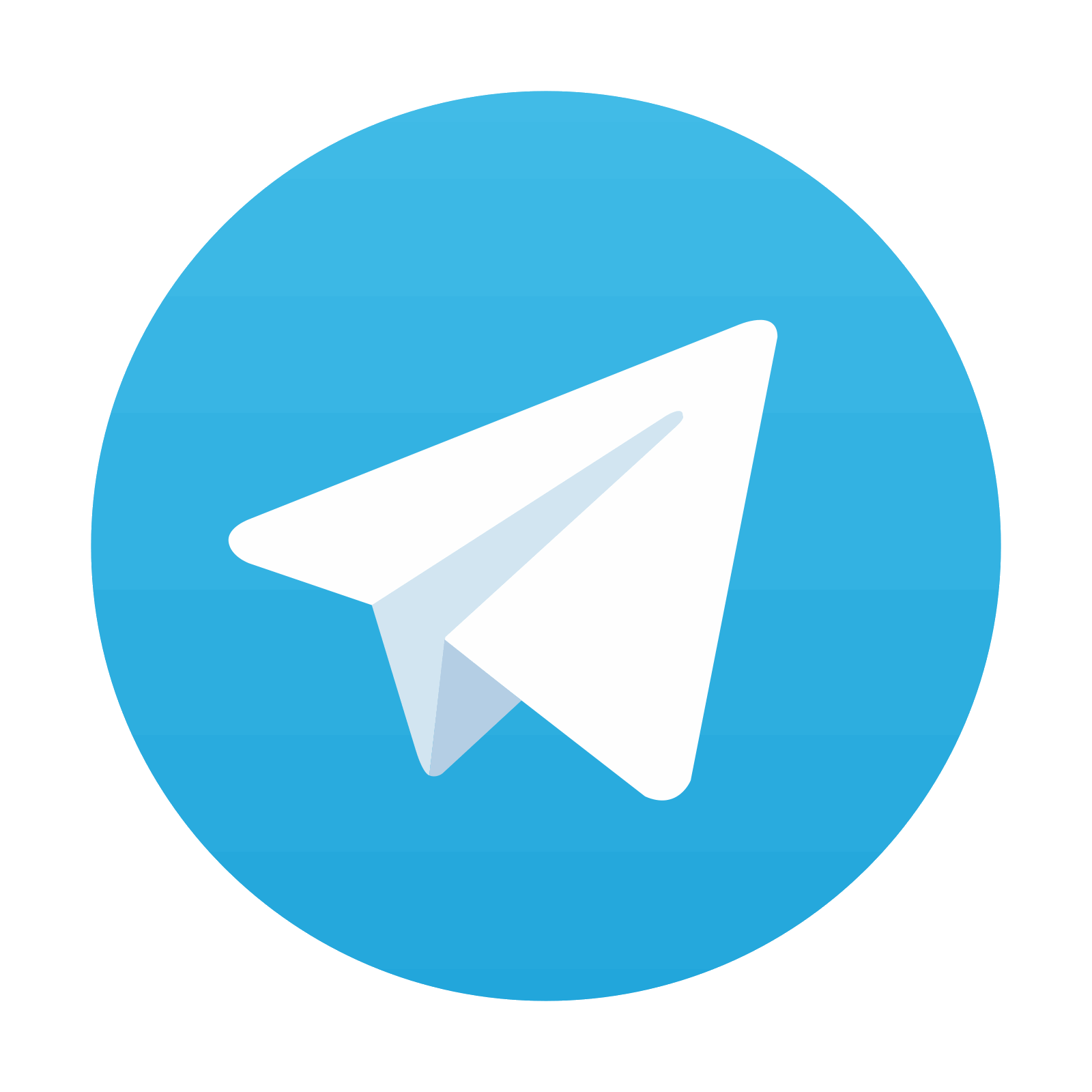
Stay updated, free articles. Join our Telegram channel
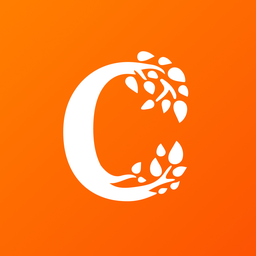
Full access? Get Clinical Tree
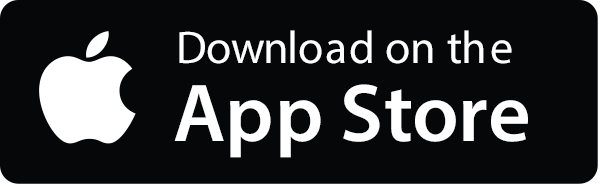
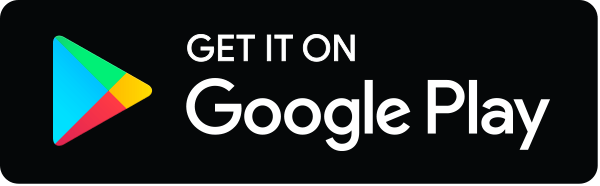
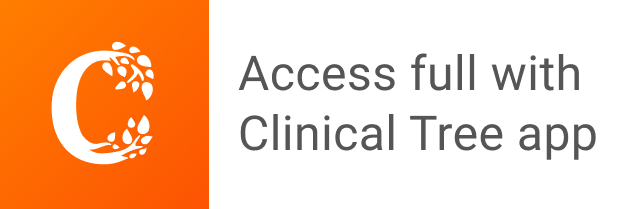