In the ICF, potassium is the dominant cation. Total body potassium is normally approximately 42 mEq/kg, and most of this potassium is intracellular and freely exchangeable. Magnesium and sodium ions also contribute to a lesser extent to the cationic component of the ICF. Phosphate, sulfate anions, bicarbonate, and intracellular proteins balance these cations.
The Gibbs–Donnan equilibrium describes the relationship between charged particles in a solution that are unevenly distributed across a semipermeable membrane.4 This special type of equilibrium exists between the ICF and ECF because of the high concentration of protein and nondiffusible phosphates in the cell. ECF cations are in electrochemical balance with chloride, bicarbonate, phosphate, and sulfate anions, although chloride is the major contributor to this balance. In addition, anionic proteins contribute to ion balance in plasma but not in interstitial fluid, which is essentially an ultrafiltrate of plasma that normally contains little protein. As a result, the content of both cations and anions in interstitial fluid is slightly higher than in plasma (Table 11-2).
Interstitial fluid, in comparison, contains little protein. These impermeable intracellular negative charges tend to favor diffusion of permeable anions into the ECF. The Gibbs–Donnan equilibrium also exists across the capillary endothelial membrane because the concentration of protein is higher on the blood side of the capillary than on the interstitial fluid side. Thus, the concentrations of diffusible ions are not necessarily equal across these membranes because of the presence of these complex anions.
Table 11-2 Electrolyte Concentrations of Intracellular and Extracellular Fluid Compartments
Osmotic Activity of Body Fluids
The concentration of solutes in the fluid compartments depends on the osmotic activity generated by the ion species contained in each compartment. When two solutions are separated by a semipermeable membrane, water moves across the membrane to equalize the concentration of osmotically active particles to which the membrane is impermeable. The maintenance of osmotic equilibrium across a semipermeable membrane is based primarily on the number of solute particles rather than on the molar concentration of the solution on each side of the membrane due to the flow of water.
The unit of measurement of osmotically active particles is the osmole (Osm) or milliosmole (mOsm) rather than the conventional units of solute concentration such as milliequivalents per liter (mEq/L). In solution, osmolarity (mOsm/L) and osmolality (mOsm/kg water) define the osmotic activity of particles. When 1 mol of NaCl dissociates in water to Na+ and Cl−, it produces 2 Osm. The same relationship holds true of dissociating salts of multivalent ions such as calcium and magnesium. One mol of a nondissociating molecule, such as glucose, produces 1 Osm (1,000 mOsm). The measured osmolality of a solution may not equal the calculated osmolality if the ions do not totally dissociate.
Body fluids are aqueous solutions composed of water and various solutes within the different body fluid compartments. Because cells are bounded by a semipermeable membrane, adding free water to the fluid surrounding a cell causes water to move across the cell membrane to equalize the osmolality differential between the intracellular and extracellular compartments. On a larger scale, adding free water to the ECF of the body causes an immediate expansion of the extracellular space, followed by a redistribution of water into the intracellular compartment (Fig. 11-1A). Conversely, loss of free water from the extracellular space ultimately leads to a shift of water from the intracellular to the extracellular space (Fig. 11-1B). An osmotic gradient of just 1 mOsm generates a pressure gradient of 19.3 mm Hg.
Colloid Oncotic Pressure (Colloid Osmotic Pressure)
Plasma proteins are confined primarily to the intravascular space and contribute to the osmotic pressure developed between the plasma and the interstitial fluid. Normal plasma protein levels of 7 g/dL contribute approximately 0.8 mOsm/L.
Figure 11-1. A: The equilibration of water from the extracellular to the intracellular space after the addition of free water to the extracellular fluid compartment. Osmolality transiently decreases in the extracellular compartment, causing water to move across the cell membranes into the intracellular space. B: Similar shifts after free water loss from the extracellular compartment. Water moves from the intracellular space to the extracellular space in response to the osmolal gradient that is established.
Osmoregulation
4 Osmolality of body fluids stays fairly constant at approximately 285 to 295 mOsm/L as the result of tightly regulated water balance. Osmoreceptor cells in the paraventricular and supraoptic nuclei of the hypothalamus exert central control over the thirst mechanism and antidiuretic hormone (ADH) secretion from the posterior pituitary.5 In the presence of excess free water, ECF osmolality falls toward 280 mOsm/kg H2O, thirst is inhibited, and ADH levels decline. In the absence of ADH, the permeability of renal collecting tubules to water is decreased, causing free water reabsorption to decrease and excretion to increase. Urine osmolality (Uosm) can decline to 50 mOsm/kg H2O (Fig. 11-2). As excess free water is eliminated, Posm begins to rise. Conversely, free water depletion causes an increase in Posm. As Posm approaches 295 mOsm/kg H2O, thirst is stimulated as is ADH secretion. As ADH levels rise to approximately 5 pg/mL, the renal collecting tubules become maximally permeable to water. Water is reabsorbed from the collecting ducts in response to the concentration gradient developed in the renal medullary interstitium. Thus, the final concentration of urine depends on both the permeability of the collecting ducts (controlled by ADH secretion) and the concentration of the medullary interstitium.5 Maximal Uosm may approach 1,200 mOsm/kg H2O. The net effect of these mechanisms is to promptly return high or low Posm to normal. The high sensitivity of the osmoreceptors and the responsiveness of the ADH feedback system ensure that even small changes in Posm result in marked alterations in urine concentration. This relation can be expressed as follows:
Urine osmolality = 95 × Plasma osmolality
Thus, a 1-mOsm change in Posm results in a 95-fold change in Uosm.
Angiotensin II and neural input from medullary baroreceptors can also influence ADH secretion and thirst, thus tying water balance to hemodynamic alterations. Relatively small changes in pressure have little effect on ADH secretion, but large decreases in pressure can cause tremendous increases in ADH release. Therefore, ADH release as a response to changes in serum osmolality is regulated by a very sensitive system (only small changes in serum osmolality outside its normal range lead to dramatic changes in ADH release), while the nonosmotic release of ADH occurs in the setting of profound hypotension for the purposes of preserving volume homeostasis with the subsequent effect of water retention regardless of serum osmolality (Fig. 11-3).
Tonicity of Body Fluids
Tonicity refers to the effect of the particles on cell volume. (NB: Osmolality defines the concentration of osmotically active particles in solution.) Permeable solutes can freely cross cell membranes, whereas impermeable solutes cannot. Although permeable solutes contribute to the osmolality of a solution, they have no effect on tonicity because they contribute neither to oncotic gradients across cell membranes nor to alterations in cell volume. Sodium is an example of an impermeable solute that affects both osmolality and tonicity. Urea and ethanol are permeable solutes that contribute to osmolality but have little effect on tonicity because they distribute equally across membranes.
Figure 11-2. The relation of plasma antidiuretic hormone (arginine vasopressin [AVP]) secretion to plasma (A) and urine (B) osmolality in healthy adults in varying states of water balance. (Reproduced with permission from Robertson GL, Berl T. Water metabolism. In: Brenner BM, Rector FC Jr, eds. The Kidney. Philadelphia, PA: WB Saunders; 1986:392.)
Figure 11-3. Effect of acute changes in blood volume or pressure on the osmoregulation of antidiuretic hormone (ADH; vasopressin). The heavy oblique line in the center represents the relation between plasma ADH and osmolality under normovolemic, normotensive conditions. The lines to the left and right show the shift in the relation when blood volume or blood pressure is acutely decreased or increased by the percentage indicated in the circles. (Reproduced with permission from Robertson GL. Physiology of ADH secretion. Kidney Int 1987;32(Suppl 21):520.)
Both impermeable and permeable solutes can contribute to hyperosmolar and hypoosmolar states. However, hypoosmolar states are always accompanied by hypotonicity, whereas hyperosmolar states are not always associated with hypertonicity. There may be marked hyperosmolality without hypertonicity with elevation of blood urea nitrogen (BUN) levels because urea is a freely permeable molecule. In contrast, elevated levels of plasma glucose in diabetic patients are associated with hyperosmolarity and associated hypertonicity. Insulin increases the transport of glucose across cell membranes, rendering these osmoles ineffective and reducing hypertonicity. Plasma hyperglycemia is associated with the movement of intracellular water to the extracellular space. This causes expansion of ECF and plasma volume and a consequent decrease in the concentration of plasma sodium. For every 100 mg/dL elevation in blood glucose, measured serum sodium is calculated to fall 1.5 mEq/L, without an actual alteration of body sodium content. The osmotic diuresis caused by the elevated glucose level tends to normalize the serum sodium if adequate hydration is maintained. Some patients with uncontrolled diabetes also have marked hyperlipidemia. Because of this, the concentration of measured sodium falls. This condition is termed pseudohyponatremia, an artifactual hyponatremia most commonly caused by severe hypertriglyceridemia, or by severe hyperproteinemia.
Plasma osmolality (Posm) is an excellent measure of total body osmolality. Osmolality differentials between fluid compartments are only transient because fluid shifts maintain isosmotic conditions. Sodium is the predominant extracellular cation; thus, estimates of Posm can be made by simply doubling the serum sodium concentration (serum [Na+]):
Posm (mOsm/L) = 2 × serum [Na+]
Because glucose and BUN may make significant contributions to Posm in certain disease states, this formula is modified for glucose and for BUN:
Posm (mOsm/L) = 2 × Serum [Na+] – Glucose/18 − BUN/2.8
Discrepancies of greater than 15 mOsm/L between calculated Posm and Posm measured in the clinical laboratory may be the result of the presence of other osmotically active particles, such as mannitol, ethanol, or ethylene glycol, or of a reduced fraction of plasma water secondary to high levels of myeloma proteins or hypertriglyceridemia.
FLUID BALANCE
Sodium Concentration and Water Balance
Abnormalities in serum sodium are usually indicative of abnormal TBW content. This is due to sodium being the primary extracellular cation. As potassium is the predominant intracellular cation, the serum [Na+] approximates the sum of the exchangeable total body sodium (Na+e) and total body exchangeable potassium (K+e) divided by TBW:
Serum [Na+] = (Na+e − K+e)/TBW
Because the sum of total body solute content (Na+e − K+e) remains relatively stable over time, changes in TBW content is, therefore, inversely proportional to changes in the serum [Na+] (Fig. 11-4).
Volume Control
5 Changes in volume (i.e., hypovolemic/nonosmotic stimuli) are detected by both osmoreceptors and baroreceptors.3 The osmoreceptors are responsible for the day-to-day fine-tuning of volume by responding to changes in tonicity, whereas the baroreceptors contribute relatively little to the control of fluid balance under normal conditions. As mentioned previously, large changes in circulating volume (10% to 20% blood volume loss) can modify the osmoregulation of ADH secretion. Cardiac atrial baroreceptors control volume by means of sympathetic and parasympathetic neural mechanisms, whereas ANP released by atrial myocytes in response to atrial wall distention may influence sodium-linked volume control by inhibition of renal sodium reabsorption.6–8
Figure 11-4. Relation between serum [Na+] and the ratio of (Na+e + K+e) to total body water (TBW). (Reproduced with permission from Edelman IS, Liebman J, O’Meara MP, et al. Interrelationships between serum sodium concentration, serum osmolarity and total exchangeable sodium, total exchangeable potassium and total body water. J Clin Invest 1958;37:1236.)
Osmoreceptors
Specialized cells in the hypothalamus that respond to changes in extracellular tonicity are known as osmoreceptors. It is believed that the activity of ion channels in the cell membrane, aquaporins, and changes in cell volume contribute to the function of these receptors. As the majority of the ECF tonicity is contributed by sodium, under normal physiologic conditions, these receptors function as “osmo-sodium” receptors. Osmoreceptors can respond to changes in tonicity as small as a few percent. In effect, these receptors monitor water balance by monitoring the tonic effect of changes in water volume. Therefore, ECF [Na+] is an effective monitor of TBW.
Baroreceptor Modulation of Volume Control
Effective circulating volume describes that portion of the extracellular volume that perfuses the organs of the body and affects the baroreceptors. The effective circulating volume normally corresponds to the intravascular volume.
Changes in the effective circulating volume are sensed by volume receptors in the intrathoracic capacitance vessels and atria, pressure receptors of the aortic arch and carotid arteries, intrarenal baroreceptors, and hepatic and cerebrospinal volume receptors.8,9 These stretch receptors respond to changes in pressure and circulating volume and, through a complex system of neural and hormonal actions, alter sodium and water balance in the kidneys. Hormonal effects are mediated by the renin–angiotensin system, aldosterone, ANP, dopamine, and renal prostaglandins.
Baroreceptor Function
The low-pressure baroreceptors of the intrathoracic vena cava and atria are located in vessels that are distensible and not affected by sympathetic stimulation; thus, they are ideally situated to detect changes in venous volume.8 These receptors send continuous signals through vagal afferent nerves to the cardiovascular control centers of the medulla and hypothalamus, which, in turn, send signals through parasympathetic and sympathetic fibers to the heart and kidneys. Changes in stretch of these vessels result in changes in the frequency of signal output from these receptors. Increases in atrial distention cause decreased nerve signal traffic, which ultimately causes increased sympathetic tone to the heart, which results in tachycardia and inhibition of sympathetic tone to the kidney. This leads to increased renal blood flow and decreased tubular sodium reabsorption. Conversely, low volume in the intrathoracic vessels results in increased sympathetic tone to the kidneys, decreased renal blood flow, and increased sodium reabsorption.
The effects of sympathetic activity on renal sodium reabsorption are probably mediated both by direct tubular innervation and by β-adrenergic stimulation of renin production. Whether this effect is crucial to fine regulation of sodium balance under normal physiologic conditions is unclear. Renal denervation in conscious, unstressed animals results in minimal alteration of either blood flow or sodium reabsorption. The effects of renal denervation become much more marked with anesthesia administration or hypotension, suggesting that sympathetic effects on renal function may be important during periods of physiologic stress.
Arterial baroreceptors are located in the aortic arch and carotid arteries. They respond to changes in heart rate, arterial pressure, and the rate of rise in the arterial pressure. Arterial baroreceptors are important during periods in which there are extremes in the changes in arterial pressure characteristics, as occur during hemorrhage. They are probably not involved in controlling subtle volume or pressure changes. In addition to large-vessel baroreceptors, there are arterial baroreceptors in the afferent arterioles of the kidneys. These baroreceptors modulate renin secretion. Increases in transmural pressure cause suppression of renin release, and decreases in transmural pressure stimulate renin release.
Hormonal Mediators of Volume Control
Renin–Angiotensin System. Renin is a proteolytic enzyme that is released from the juxtaglomerular cells of afferent arterioles in the kidney in response to several stimuli. These include changes in arterial pressure, changes in sodium chloride delivery to the macula densa of the distal convoluted tubule, increases in β-adrenergic activity, and increases in cellular cyclic adenosine monophosphate.
Renin cleaves angiotensin I from circulating angiotensinogen, produced by the liver. Angiotensin I is cleaved to the octapeptide angiotensin II by angiotensin-converting enzyme (ACE), which is produced by vascular endothelial cells. One pass through the pulmonary microvasculature converts most angiotensin I to angiotensin II. Angiotensin II acts both locally and systemically to increase vascular tone. It also stimulates catecholamine release from the adrenal medulla, increases sympathetic tone through central effects, and stimulates catecholamine release from sympathetic nerve terminals. Angiotensin II affects sodium reabsorption by decreasing renal blood flow and glomerular filtration. This results in altered tubuloglomerular feedback, the mechanism by which changes in distal tubular NaCl delivery alter glomerular blood flow. Finally, angiotensin II increases sodium reabsorption by direct tubular action as well as by stimulation of aldosterone release from the adrenal cortex. The multiplicity of actions of angiotensin is depicted in Figure 11-5.
Aldosterone. Aldosterone is a mineralocorticoid produced in the zona glomerulosa of the adrenal cortex. Aldosterone increases renal tubular reabsorption of sodium. Aldosterone acts directly on the distal tubule cells by modifying gene expression and stabilizing the epithelial Na+ channel in the open state and by increasing the number of channels in the apical membrane of these cells.5 By increasing protein production in these tubular cells, aldosterone induces an influx of sodium, which causes an increase in cellular Na+-K+-adenosine triphosphatase activity. The net result is increased sodium reabsorption and the obligate loss of potassium via the renal outer medullary potassium (ROMK) channel. Although the primary regulator of aldosterone secretion is angiotensin II, aldosterone release is also stimulated by increased potassium levels, adrenocorticotropic hormone, endothelins, and prostaglandins.10
Figure 11-5. Multiple effects of increased angiotensin II release in response to the stimulus of decreased extracellular volume.
Atrial and Renal Natriuretic Peptides. ANP is synthesized and released by atrial myocytes in response to atrial wall distention. As mentioned previously, small changes in right atrial pressure produce large increases in plasma levels of ANP.7,8 There is evidence that ANP has a direct inhibitory effect on renal sodium reabsorption, which is probably maximal at the level of the medullary collecting tubules. Although pharmacologic doses of ANP can cause changes in both renal blood flow and glomerular filtration rate (GFR), physiologic levels do not appear to have any major effect on these parameters. Other active fragments of the ANP prohormone have been found to have natriuretic activity. The best described is urodilatin, also known as renal natriuretic peptide. Urodilatin is a peptide with ANP-like activity that was first isolated from human urine. It is synthesized and luminally secreted by cortical collecting tubule cells. Like ANP, it is released in the kidney tubules in response to atrial distention and saline loading. It is at least twice as potent as ANP, acting in the distal nephron to cause a rise in intracellular cyclic guanosine monophosphate, leading to sodium, chloride, and water diuresis. ANP and other peptides may play an important role in controlling intravascular volume and water and electrolyte secretion.
Renal Prostaglandins. Renal prostaglandins appear to play a role in volume control, although under normal physiologic conditions, this role may be minimal. Disease states such as sepsis and jaundice, or the induction of anesthesia, may make the contribution of the prostaglandins more pronounced.9
Prostaglandin E2 (PGE2) and prostaglandin I2 (PGI2) appear to be the predominant prostaglandins produced in the kidney. PGE2 is produced primarily by the interstitial cells of the renal medulla. The release of PGE2 has been shown to depend on increases in interstitial pressure, which can be induced by changes in renal perfusion, ureteral obstruction, or alterations in oncotic pressure. Under these conditions, PGE2 increases sodium excretion in the absence of changes in GFR. PGE2 antagonizes the action of vasopressin (ADH) and inhibits ADH-induced sodium reabsorption along the medullary collecting duct and thick ascending limb. PGI2 is produced by the glomeruli and endothelial cells of the kidney and is present in the greatest concentrations in the renal cortex. PGI2 is a vasodilator, and its effects on renal vascular resistance increase both renal blood flow and GFR. PGI2 production is augmented by increases in angiotensin, catecholamines, and sympathetic tone and may act to counterbalance their vasoconstricting effects. Although under normal physiologic conditions inhibition of prostaglandin production has little effect on renal function, administration of nonsteroidal anti-inflammatory agents, which inhibit cyclooxygenase, to patients with conditions known to cause renal dysfunction (e.g., cirrhosis) can precipitate renal failure, presumably because of loss of the protective effects of the renal prostaglandins.
Endothelins. Endothelins are peptide vasoconstrictors that are involved in volume and pressure regulation.10 Endothelin is produced and released by endothelial and other cells to act on adjacent smooth muscle cells. In addition to increasing peripheral resistance, endothelin infusion has a direct inotropic effect on the myocardium. In contrast to its vasoconstrictive effects, endothelin stimulates the release of other vasoactive mediators, particularly endogenous vasodilators like nitric oxide, which act to limit its intense vasoconstrictor effect.
Endothelin exerts a complex influence on sodium and water exchange through varied interactions with many other hormones that govern fluid and electrolyte balance. One net effect of endothelin is a decrease in the filtered load of sodium in the kidney. This results in inhibition of water reabsorption and decreased sodium excretion. Endothelin increases ANP secretion, activates ACE, and inhibits renin release by the juxtaglomerular apparatus. At low doses, endothelin-1 produces natriuresis and diuresis. Endothelin also modulates the biosynthesis of aldosterone, thereby inhibiting water reabsorption through aldosterone-controlled mechanisms. Vasopressin-mediated water reabsorption is also inhibited. Endothelin appears to have complex interactions with other regulators of renal perfusion and handling of water and electrolytes, which has stimulated research to evaluate the contribution of endothelin to the pathophysiology of various renal diseases.11
Nitric Oxide. Nitric oxide is a short-lived free radical produced from l-arginine by nitric oxide synthases.11 This substance has numerous biologic functions, including regulation of vascular tone and tissue blood flow. Nitric oxide is produced in renal smooth muscle cells, mesangial cells, tubules, and endothelial cells and participates in the regulation of renal hemodynamics and renal handling of water and electrolytes. Nitric oxide and PGI2 each independently cause renal vasodilation in response to a variety of stimuli. Nitric oxide contributes to tubuloglomerular feedback, which modulates the delivery and reabsorption of sodium and chloride in the renal tubules. Nitric oxide also regulates renin release by the juxtaglomerular apparatus. Nitric oxide produced in the proximal tubule may mediate the effects of angiotensin on tubular reabsorption.11
Normal Water and Electrolyte Exchange
Surgical patients are prone to fluid and electrolyte abnormalities. It is important to recognize that disease states, trauma, and stress-related abnormalities – especially those caused by a surgical procedure – alter many of the body’s fundamental homeostatic mechanisms. It is therefore important to understand normal fluid and electrolyte balance to avoid additional iatrogenic perturbations of these mechanisms.
Normal Water Exchange
6 Water losses are both sensible (measurable) and insensible (unmeasurable). Measurable losses include urinary and intestinal. Table 11-3 summarizes the normal sensible and insensible losses encountered in a 24-hour period. The volumes of these losses may vary considerably especially in disease states. Under normal conditions, the minimal amount of water needed to excrete normal metabolic waste products is approximately 300 to 500 mL/day.
The GI tract has a net secretory action down to the level of the jejunum. After that, the resorptive capacity of the remainder of the small and large bowel acts to keep further water loss to a minimum. Fecal water loss is usually trivial: ∼150 mL/day. In disease conditions like bowel obstruction, severe diarrhea, and enterocutaneous fistulas, GI losses of water and electrolytes can be significant causing secondary pathophysiologic conditions.
Table 11-3 Body Fluid Compartments
Sweating and diaphoresis are active processes involving the secretion of a hypotonic mixture of electrolytes and water. Sweating functions to allow the convective dissipation of heat. Diaphoresis is a similar effect, but is a pathophysiologic state stemming from a disease process.
These aforementioned conditions are distinct from the immeasurable, evaporative loss of water from the skin under normal conditions (Table 11-3). Evaporative skin losses are increased with an increase in body surface area (especially infants and small children), patient temperature, and the relative humidity of the environment. Evaporation through the skin functions through convective heat loss and is proportional to calories expended. Approximately 30 mL of water is lost for every 100 kcal expended. Respiratory exchange depends on ambient temperature, relative humidity, and on the amount of air flow. Overall, normal insensible water losses average approximately 8 to 12 mL/kg/day. Insensible water loss increases 10% for each degree of body temperature above 37.2°C (99°F). In addition, patients who breathe unhumidified air lose additional free water. Conversely, patients who are on respirators or who breathe air that is 100% humidified have no respiratory losses and may gain free water.
FLUID AND ELECTROLYTE THERAPY
Parenteral Solutions
Crystalloids
7 The most widely used parenteral solutions are composed most commonly of sodium and chloride. They are collectively referred to as crystalloids (based on the solid, room temperature form of the primary ingredient sodium chloride). Crystalloids are inexpensive and highly effective for fluid maintenance and rapid volume replacement. They also have excellent safety profiles. Although crystalloid-induced hypercoagulability has been reported, its causes are multifactorial and poorly understood.12–15 Clinically, this does not represent a significant problem.
The most commonly used crystalloid solutions available for parenteral administration are found in Table 11-4. Selection of the appropriate fluid requires assessment of the patient’s maintenance fluid needs, existing fluid deficits and anticipated ongoing fluid losses. When a single solution does not accurately replace the required electrolyte components, bolus administration of specific replacement solutions is commonly performed. It is commonplace to use more than one type of crystalloid solution. This is commonly seen with parenteral medication formulations that may have specific carrier-fluid requirements. Ions such as potassium, magnesium, or calcium may be necessary and can be added to parenteral solutions to suit the patient’s requirements.
Table 11-4 Electrolyte Content of Commonly Used Intravenous Crystalloid Solutions
Isotonic saline (0.9% sodium chloride or normal saline) contains 154 mEq of both sodium and chloride. Although this solution can be useful in patients with hyponatremia or hypochloremia, the excess of both sodium and chloride can lead to acid–base disturbances. For example, infusion of large volumes of 0.9% saline can lead to a hyperchloremic metabolic acidosis. In addition, the pH of normal saline and of the related solutions (0.45%, 0.33%, and 0.2% saline) is 4.0 to 5.0 further decreasing the blood pH.
Lactated Ringer solution is a buffered solution composed of the conjugate-base lactate. It was created in the 1930s by an American pediatrician, Alexis Hartmann, as a treatment for metabolic acidosis. The solution has a lower sodium and chloride content (130 mEq/L) and adds a very small amount of potassium and calcium (4 mEq/L each). It is ideal for the replacement of acute fluid losses where serum electrolyte concentrations are initially normal. Normal renal function usually ensures that any extra free water from this solution is excreted. Hyponatremia can occur with extended use of lactated Ringer solution. Furthermore, the presence of potassium in lactated Ringer solution has been a concern when used in patients with acute kidney injury, although more recent data has suggested that normal saline (with no potassium in solution) may actually be more likely to cause hyperkalemia in this population due to metabolic acidosis-induced cellular shifts of potassium from the cell. Although the lactate anion in lactated Ringer solution is metabolized to bicarbonate in the liver, this does not appear to contribute to acidosis in normal hepatic function. Work comparing the D,L-racemic mixture of lactate has implicated the D-isomer in leukocyte activation.16 There are no compelling data to suggest that resuscitation with this solution increases the inflammatory response. Utilization of only the L-isomer is presumed to eliminate this concern.16 Ringer solution is not used as a diluent in blood transfusions given the concern of calcium being chelated by citrate (an anticoagulant in blood products), which may promote clotting in donor blood.
For maintenance fluid therapy especially prior to an operation with nil per os status or following correction of any existing deficits, less concentrated saline solutions are more appropriate to replace ongoing fluid losses (e.g., nasogastric tube losses). The specific crystalloid selected is best determined by calculated requirements. These fluids are hypoosmotic and hypotonic with respect to plasma. In theory, rapid infusion of very hypotonic solutions can result in red blood cell lysis. For this reason, 5% dextrose (50 g of dextrose per liter) has traditionally been added to these solutions to increase tonicity. The addition of dextrose is beneficial in pediatric patients who are unable to adequately control glucose homeostasis due to immature livers. A 5% dextrose solution represents 200 kcal per liter of solution.
Hypertonic saline solutions (HTSs) (3% NaCl and 5% NaCl) are generally used to replace sodium deficits in patients with symptomatic hyponatremia. These and even more concentrated (7.5% and 23% NaCl) solutions have also been used for resuscitation of hemorrhagic shock, head trauma, and burn patients.17–19 Hypertonic saline appears to increase intravascular volume in these patients more quickly than isotonic solutions. It is believed that the osmotic gradient produced redistributes fluids from the perivascular and intracellular spaces to the intravascular space with consequent plasma volume expansion (up to fourfold). This, in turn, may decrease the total resuscitation volume requirement. When HTS is used for resuscitation of patients with severe sepsis, it has been shown to result in improvements in oxygen transport, cardiac output, and pulmonary capillary wedge pressure.17 The systemic and mesenteric oxygen extraction coefficient improves without worsening of other markers of perfusion. This is achieved by rapid mobilization of fluids from the intracellular compartment to the extracellular compartment. These cardiovascular and hemodynamic effects are short-lived, in general lasting from 60 to 120 minutes.
The osmotic effect of hypertonic saline may benefit patients with acute severe brain injury.19 By reducing the water content of the brain, HTS can help to control intracerebral pressure after injury. In addition, HTS has immunomodulatory effects, which are well documented.20 HTS reduces the systemic inflammatory response syndrome and may attenuate multiple organ dysfunction syndrome. HTSs are not without adverse effects, however. Patients are at risk for electrolyte abnormalities such as hypernatremia, hyperchloremia, and consequent metabolic acidosis. Extravasation into the soft tissues can produce significant soft tissue edema and even necrosis.
Colloids
Colloids are composed of large molecules (e.g., albumin) that have a greater tendency to stay in the intravascular space. They appear to be more effective at enhancing the plasma volume than do crystalloid fluids. Colloid solutions offer the potential benefits of promoting fluid retention in the intravascular space and reducing excess interstitial fluid (edema). Worldwide, albumin and artificial colloids are relied on to a varying degree in fluid management of the surgical patient. In the United States, concerns regarding the effectiveness, cost, and potential complications of colloid administration have limited their use to specific clinical situations.
Albumin
Albumin (69 kD) is the primary protein in plasma responsible for oncotic pressure. Exogenous human albumin is derived from human plasma and heat-treated to reduce the risk of infection. It is available in a 5% (50 g/L) and a 25% solution (250 g/L) in isotonic saline. These act by increasing plasma oncotic pressures and theoretically slowing or even reversing movement of water into the interstitial space. Albumin has an approximate intravascular half-life of 4 hours in conditions of normal physiological capillary permeability. However, many of the conditions associated with edema are also associated with abnormalities in microvascular permeability (e.g., systemic inflammatory disease). For example, the pulmonary circulation in the adult respiratory distress syndrome, regional circulatory beds in postoperative patients, burns or infections, and the systemic circulation in sepsis all lead to conditions resulting in increased microvascular permeability. It is, therefore, believed that exogenously administered protein in colloid solutions quickly extravasate into the interstitial space and paradoxically intensify – rather than decrease – interstitial edema. The SAFE trial (Saline vs. Albumin Fluid Evaluation) found no overall difference in organ dysfunction or survival when comparing saline administration to albumin infusion.21 However, the SOAP trial (Sepsis Occurrence in Acutely Ill Patients) demonstrated increased mortality in intensive care unit patients treated with colloids.22,23 As mentioned above, albumin solutions are heat-treated to negate viral and bacterial contamination. Allergic reactions are rare. The primary concern with albumin administration is cost; the infection risk is negligible.
Hetastarch
Hydroxyethyl starches (HES) are synthetic colloids derived from hydrolyzed amylopectin. In the United States, it is available as a 6% solution of high–molecular-weight (150 to 450 kD), highly hydroxyethyl substituted HES in normal, isotonic saline. The average molecular weight of the starch molecules is equivalent to that of albumin. However, HES is slightly more effective than 5% albumin as a colloid – it has a higher colloid oncotic pressure than 5% albumin. The main advantage of hetastarch is its lower cost compared to albumin.
Hetastarch has been used as a resuscitative solution in a variety of clinical settings with variable results. Coagulopathy and bleeding complications have been widely reported after administration of highly substituted, high–molecular-weight HES.24,25 This appears to be associated with reduced factor VIII and von Willebrand factor levels, a prolonged partial thromboplastin time, and impaired platelet function.26 It also has a long elimination half-life (17 days). Because of its long half-life, the coagulopathy does not rapidly reverse after cessation of HES administration. Also, serum amylase enzymes continually degrade HES molecules before renal clearance. It is common for serum amylase levels to be elevated for the first few days after HES infusion. In order to differentiate this from pancreatitis, serum lipase levels should be checked and are usually normal.
The above observations and reports of renal insufficiency after HES administration prompted a prospective study, with results published in 2012.27,28 The results of these trials were so significant that the FDA and the Pharmacovigilance Risk Assessment Committee, the European equivalent of the FDA, recommended suspending marketing authorization of all HES products and issued warnings to its use.29,30 The Surviving Sepsis Campaign recommends against HES administration in septic patients.31
Dextrans
First introduced in the 1940s, dextrans are glucose polymers synthesized by Leuconostoc mesenteroides bacteria. These are available as synthetic plasma expanders in both 40 kD (dextran 40) and 70 kD (dextran 70) solutions. Although neither is used frequently for volume expansion, dextran 70 has been preferred because of its significantly longer half-life and better retention in plasma. It is degraded to glucose and water by cells. The use of dextrans involves risks of anaphylaxis, hyperglycemia, renal dysfunction and failure, coagulopathy (e.g., erythrocyte and platelet dysfunction), and increased thrombosis rates. The use of dextran solutions is no longer favored given a higher relative risk of mortality compared to HESs.32
Gelatins
Gelatin solutions produced from bovine collagen are effective as plasma volume expanders. These are currently only available outside the United States in urea-linked (Gelofusin) and succinate-linked (Haemaccel) formulations. Gelofusin has been used in similar clinical settings to HES, with similar clinical results.33 Uniquely, coagulopathy does not appear to be an issue with gelatins. Renal impairment has been reported with these colloids as having allergic reactions ranging in severity from pruritus to anaphylaxis with both gelatin formulations.34
Artificial Oxygen Carriers
Recent clinical trials and subgroup analyses using temporary hemoglobin-based oxygen-carrying solutions (e.g., PolyHeme, Northfield Laboratories, Inc.) have been encouraging albeit with some controversy.35 PolyHeme is made from polymerized human hemoglobin. It originally began as a military project following the Vietnam war. PolyHeme’s primary use is in trauma situations with massive blood loss when fresh, whole blood is not readily available for transfusion. On May 9, 2009, Northfield Laboratories, Inc. shut down operations after it failed to secure a biologic license application for PolyHeme from the FDA. However, with further studies, solutions like PolyHeme may prove beneficial in the future when volume expansion in conjunction with increased oxygen-carrying capacity is required.
GOALS OF FLUID AND ELECTROLYTE THERAPY
Maintaining homeostasis – body fluid and electrolyte concentrations – and normal hemodynamic parameters are the goals of fluid and electrolyte therapy. This is accomplished by first understanding the general maintenance fluid required by an idealized 70-kg male patient to account for normal daily losses. Thereafter, it is imperative to correct existing volume and electrolyte abnormalities and any ongoing losses associated with disease states and surgical treatments.
Table 11-5 Calculation of Maintenance Fluid Requirements
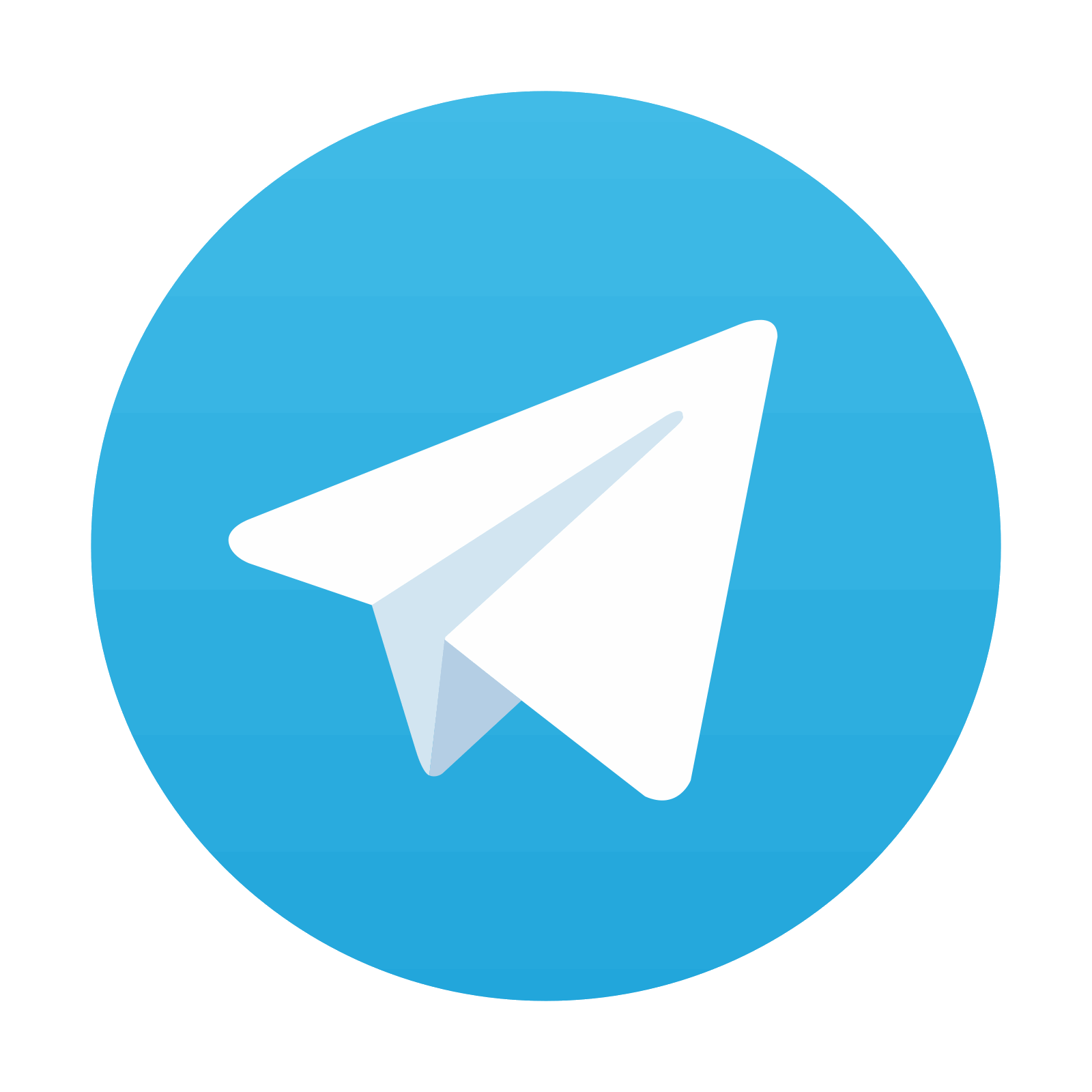
Stay updated, free articles. Join our Telegram channel
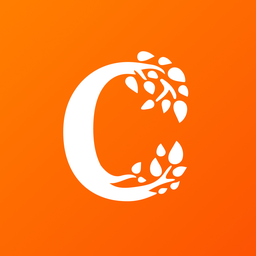
Full access? Get Clinical Tree
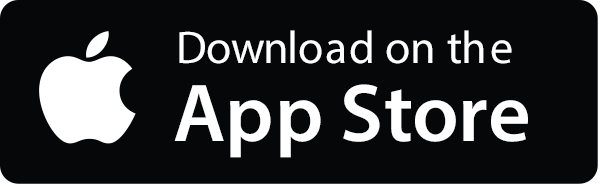
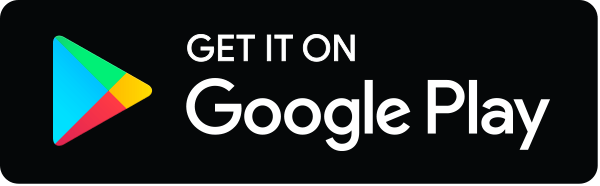