The Fasting State. Fasting begins approximately 2 to 4 hours after a meal, when blood glucose levels return to basal levels, and continues until blood glucose levels begin to rise after the start of the next meal. Within about 1 hour after a meal, blood glucose levels begin to fall. Consequently, insulin levels decline and glucagon levels rise. These changes in hormone levels trigger the release of fuels from the body stores. Liver glycogen is degraded by the process of glycogenolysis, which supplies glucose to the blood. Adipose triacylglycerols are mobilized by the process of lipolysis, which releases fatty acids and glycerol into the blood. Use of fatty acids as a fuel increases with the length of the fast; they are the major fuel oxidized during overnight fasting.
Fuel Oxidation. During fasting, glucose continues to be oxidized by glucose-dependent tissues such as the brain (nervous system tissues) and red blood cells, and fatty acids are oxidized by tissues such as muscle and liver. Muscle and most other tissues oxidize fatty acids completely to CO2 and H2O. However, in an extended fast, the liver partially oxidizes fatty acids to smaller molecules called ketone bodies, which are released into the blood. Muscle, kidney, and certain other tissues derive energy from completely oxidizing ketone bodies in the tricarboxylic acid (TCA) cycle.
Maintenance of Blood Glucose. As fasting progresses, the liver produces glucose not only by glycogenolysis (the release of glucose from glycogen) but also by a second process called gluconeogenesis (the synthesis of glucose from noncarbohydrate compounds). The major sources of carbon for gluconeogenesis are lactate, glycerol, and amino acids. When the carbons of the amino acids are converted to glucose by the liver, their nitrogen is converted to urea.
Starvation. When we fast for 3 or more days, we are in a starved state. Muscle continues to burn fatty acids but decreases its use of ketone bodies. As a result, the concentration of ketone bodies rises in the blood to a level at which the brain begins to oxidize them for energy. The brain then needs less glucose, so the liver decreases its rate of gluconeogenesis. Consequently, less protein in muscle and other tissues is degraded to supply amino acids for gluconeogenesis. Protein sparing preserves vital functions for as long as possible. Because of these changes in the fuel utilization patterns of various tissues, humans can survive for extended periods without ingesting food.
THE WAITING ROOM 
Percy V. had been admitted to the hospital with a diagnosis of depression related to bereavement and malnutrition (see Chapter 1). At the time of admission, his body weight of 125 lb gave him a body mass index (BMI) of 17.5 (healthy range, 18.5 to 24.9). His serum albumin was 10% below the low end of the normal range, and he exhibited signs of iron and vitamin deficiencies.
Additional tests were done to help evaluate Percy V.’s degree of malnutrition and his progress toward recovery. His arm circumference and triceps skinfold were measured, and his mid-upper arm muscle circumference (MUAMC) was calculated (see Chapter 2, Anthropometric Measurements). His serum prealbumin and his serum albumin were measured. Fasting blood glucose and serum ketone body concentration were determined on blood samples drawn the next day before breakfast. A urine specimen was collected to determine ketone body excretion, and a 24-hour creatinine excretion can be used for calculation of the creatinine–height index (CHI), a measure of protein depletion from skeletal muscle.
Ann R. was receiving psychological counseling for anorexia nervosa, but with little success (see Chapter 1). She continues to want to lose weight despite being underweight. She saw her gynecologist because she had not had a menstrual period for 5 months. She also complained of becoming easily fatigued. The physician recognized that Ann R.’s body weight of 85 lb was now <65% of her ideal weight, and he calculated that her BMI was now 13.7. The physician recommended immediate hospitalization. The admission diagnosis was severe malnutrition (protein–energy malnutrition) secondary to anorexia nervosa. Clinical findings included decreased body core temperature, blood pressure, and pulse (adaptive responses to malnutrition). Her physician ordered measurements of blood glucose as well as other blood tests to check electrolytes and her kidney function, and an electrocardiogram to see if there were any effects on the electrical activity of the heart.
I. The Fasting State
Blood glucose levels peak about 1 hour after eating (the postprandial state) and then decrease as tissues oxidize glucose or convert it to storage forms of fuel. By 2 hours after a meal, the level returns to the fasting range (between 70 and 100 mg/dL). This decrease in blood glucose causes the pancreas to decrease its secretion of insulin, and the serum insulin level decreases. The liver responds to this hormonal signal by starting to degrade its glycogen stores (glycogenolysis) and release glucose into the blood.
If we eat another meal within a few hours, we return to the fed state. However, if we continue to fast for 12 hours, we enter the basal state (also known as the postabsorptive state). A person is generally considered to be in the basal state after an overnight fast when no food has been eaten since dinner the previous evening. By this time, the serum insulin level is low and glucagon is rising. Figure 3.1 illustrates the main features of the basal state.
FIGURE 3.1 Basal state. This state occurs after an overnight (12-hour) fast. The circled numbers serve as a guide indicating the approximate order in which the processes begin to occur. It is important to note that certain amino acids can also give rise to acetyl CoA (not just glucose). AA, amino acid; ATP, adenosine triphosphate; acetyl CoA, acetyl coenzyme A; FA, fatty acids; KB, ketone bodies; RBC, red blood cells; TCA, tricarboxylic acid; TG, triacylglycerols.
A. Blood Glucose and the Role of the Liver during Fasting
The liver maintains blood glucose levels during fasting, and its role is thus critical. Glucose is the major fuel for tissues such as the brain and neural tissue, and the sole fuel for red blood cells. Most neurons lack enzymes required for the oxidation of fatty acids, but they can use ketone bodies to a limited extent. Red blood cells lack mitochondria, which contain the enzymes of fatty acid and ketone body oxidation, and can use only glucose as a fuel. Therefore, it is imperative that blood glucose not decrease too rapidly nor fall too low.
Lactate is a product of glycolysis in red blood cells and exercising muscle; glycerol is obtained from lipolysis of adipose triacylglycerols; and amino acids are generated by the breakdown of protein. Because our muscle mass is so large, most of the amino acids are supplied from the degradation of muscle protein. These compounds travel in the blood to the liver, where they are converted to glucose by gluconeogenesis. Because the nitrogen of the amino acids can form ammonia, which is toxic to the body, the liver converts this nitrogen to urea. Urea has two amino groups for just one carbon (Fig. 3.2). It is a very soluble, nontoxic compound that can be readily excreted by the kidneys and thus is an efficient means for disposing of excess ammonia.
FIGURE 3.2 The structure of urea, the highly soluble nitrogen disposal molecule.
As fasting progresses, gluconeogenesis becomes increasingly more important as a source of blood glucose. After a day or so of fasting, liver glycogen stores are depleted and gluconeogenesis is the only source of blood glucose.
B. Role of Adipose Tissue during Fasting
As blood insulin levels decrease and blood glucagon levels rise, adipose triacylglycerols are mobilized by a process known as lipolysis (lysis of triacylglycerol). They are converted to fatty acids and glycerol, which enter the blood.
It is important to realize that it is only the glycerol and not the fatty acid component of triacylglycerols that provides the carbon for gluconeogenesis. Thus, of the vast store of food energy in adipose tissue triacylglycerols, only the small glycerol portion travels to the liver to enter the gluconeogenic pathway.
Fatty acids serve as fuel for muscle, kidney, and most other tissues. They are oxidized to acetyl coenzyme A (acetyl CoA), and subsequently to CO2 and H2O in the TCA cycle, producing energy in the form of adenosine triphosphate (ATP). In addition to the ATP required to maintain cellular integrity, muscle uses ATP for contraction and the kidney uses ATP to drive its transport of metabolites across the nephron membrane.
The fatty acids that enter the liver are converted to acetyl CoA prior to being completely oxidized to CO2 via the Krebs TCA cycle. The process of conversion of fatty acids to acetyl CoA (β oxidation) produces a considerable amount of energy (ATP), which drives the reactions of the liver under these conditions. The major ATP-requiring process occurring in the liver at this time is gluconeogenesis. The acetyl CoA derived from fatty acid oxidation can also be converted to the ketone bodies acetoacetate and β-hydroxybutyrate, which are released into the blood (Fig. 3.3).
FIGURE 3.3 The ketone bodies β-hydroxybutyrate, acetoacetate, and acetone. β-hydroxybutyrate and acetoacetate are formed in the liver. Acetone is produced by nonenzymatic decarboxylation of acetoacetate. However, acetone is expired in the breath and is not metabolized to a significant extent in the body, whereas β-hydroxybutyrate and acetoacetate are utilized by muscle and the nervous system as an energy source.
The liver lacks an enzyme required for ketone body oxidation. However, ketone bodies can be further oxidized by most other cells with mitochondria, such as muscle and neurons. In these tissues, acetoacetate and β-hydroxybutyrate are converted to acetyl CoA and then oxidized in the TCA cycle, with subsequent generation of ATP.
C. Summary of the Metabolic Changes during a Brief Fast
In the initial stages of fasting, stored fuels are used for energy (see Fig. 3.1). The liver plays a key role in maintaining blood glucose levels in the range of 70 to 100 mg/dL, first by glycogenolysis (Fig. 3.1, circle 2) and subsequently by gluconeogenesis (Fig. 3.1, circles 9, 11, and 12). Lactate, glycerol, and amino acids serve as carbon sources for gluconeogenesis. Amino acids are supplied by muscle (via proteolysis, lysis of proteins to individual amino acids). Their nitrogen is converted to urea in the liver (Fig. 3.1, circle 10), which is excreted by the kidneys.
Fatty acids, which are released from adipose tissue by the process of lipolysis (Fig. 3.1, circle 5), serve as the body’s major fuel during fasting. The liver oxidizes its fatty acids to acetyl CoA. Some of the acetyl CoA is utilized for the production of ketone bodies (Fig. 3.1, circle 7), which are released into the blood. Thus, during the initial stages of fasting, blood levels of fatty acids and ketone bodies begin to increase. Muscle uses fatty acids, ketone bodies (Fig. 3.1, circles 6 and 8), and (when exercising and while supplies last) glucose from muscle glycogen. Many other tissues use either fatty acids or ketone bodies. However, red blood cells, the brain, and other neural tissues use mainly glucose (Fig. 3.1, circles 3 and 4). The metabolic capacities of different tissues with respect to pathways of fuel metabolism are summarized in Table 3.1.
TABLE 3.1 Metabolic Capacities of Various Tissues
Acetyl CoA, acetyl coenzyme A; TCA, tricarboxylic acid.
++ indicates use of the fuel; +++ indicates maximal use, whereas + indicates minimal use. – indicates no use of the fuel.
II. Metabolic Changes during Prolonged Fasting
If the pattern of fuel utilization that occurs during a brief fast were to persist for an extended period, the body’s protein would be quite rapidly consumed to the point at which critical functions would be compromised. Fortunately, metabolic changes occur during prolonged fasting that conserves (spare) muscle protein by causing muscle protein turnover to decrease. Figure 3.4 shows the main features of metabolism during prolonged fasting (starvation).
FIGURE 3.4 Starved state. Broken blue lines indicate processes that have decreased, and the heavy red solid line indicates a process that has increased relative to the fasting state. As with Figure 3.1 certain amino acids can also give rise to acetyl CoA, which the liver can use for energy, but not for glucose synthesis. AA, amino acid; acetyl CoA, acetyl coenzyme A; ATP, adenosine triphosphate; FA, fatty acids; KB, ketone bodies; RBC, red blood cells; TCA, tricarboxylic acid; TG, triacylglycerols.
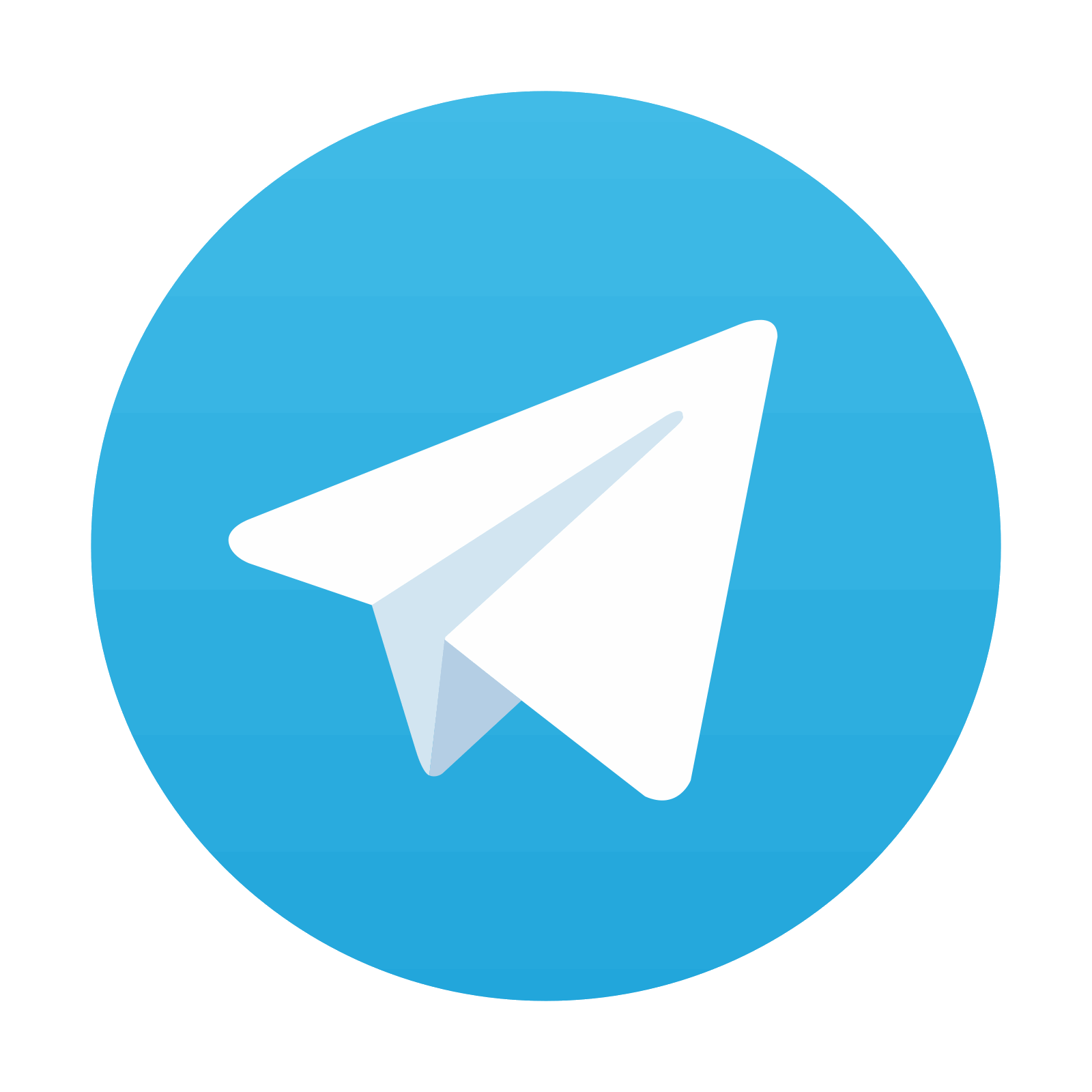
Stay updated, free articles. Join our Telegram channel
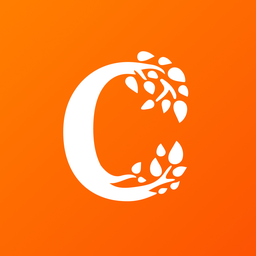
Full access? Get Clinical Tree
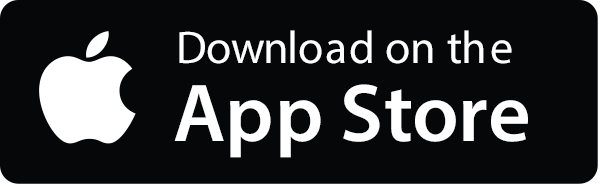
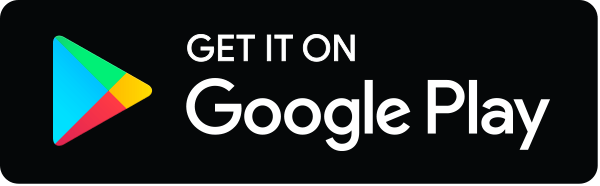