Fascia and biomechanical regulation
While everyone learns something about bones and muscles, the origin and disposition of the fascinating fascial net that unites them is less widely understood (Fig. 1.1). Although this situation is changing rapidly as increased research broadens our knowledge,1 the vast majority of the public – and even most therapists and athletes – still base their thinking about their own structure and movement on the limited idea that there are individual muscles that attach to bones that move us around via mechanical leverage. As Schultz and Feitis put it:
Fig. 1.1 (A) A fresh-tissue specimen of the myofascial meridian termed the Superficial Back Line, dissected intact by Todd Garcia from the Laboratories of Anatomical Enlightenment. (Photo courtesy of the author.) (This specimen is explained on video on the accompanying website.)
(B) A dissection of teased muscle fibers, showing surrounding and investing endomysial fascia. (Reproduced with kind permission from Ronald Thompson.) (DVD ref: This and other graphics are available and explained in Fascial Tensegrity, available from www.anatomytrains.com)
(C) A section of the thigh, derived from the National Library of Medicine’s Visible Human Project, using National Institute of Health software, by structural practitioner Jeffrey Linn. This gives us the first glimpse into what the fascial system would look like if that system alone were abstracted from the body as a whole. Once this process is complete for an entire body, we will have a powerful new anatomical rendering of the responsive system that handles, resists and distributes mechanical forces in the body. (Reproduced with kind permission from Jeffrey Linn.) (DVD ref: This and other graphics are available and explained in Fascial Tensegrity, available from www.anatomytrains.com)
(D) A diagram of the fascial microvacuole sliding system between the skin and the underlying tendons as described by Dr J. C. Guimberteau. (Diagram with kind permission of Dr JC Guimberteau, Plastic and Hand Surgeon and Endovivo Productions.) (DVD ref: Strolling Under the Skin, available from www.anatomytrains.com)
The muscle–bone concept presented in standard anatomical description gives a purely mechanical model of movement. It separates movement into discrete functions, failing to give a picture of the seamless integration seen in a living body. When one part moves, the body as a whole responds. Functionally, the only tissue that can mediate such responsiveness is the connective tissue.2
DVD ref: The arguments made in this chapter are summarized in less detail on: Fascial Tensegrity, available from www.anatomytrains.com.
Please note that this chapter presents a point of view, a particular set of arguments that build toward the Anatomy Trains concept, and is by no means the complete story on the roles or significance of fascia. Here, we go long on geometry, mechanics, and spatial arrangement, and drastically short on chemistry. We concern ourselves with the healthy supporting role of fascia in posture and movement, totally avoiding any discussion of pathology. Other more diverse and excellent descriptions are referenced here for the interested reader; the more clinically minded may wish to skip this antipasti and go straight on to the main course which begins in Chapter 3.
‘Blessed be the ties that bind’: fascia holds our cells together
Life on this planet builds itself around a basic unit – the cell. Although we can easily imagine great globs of undifferentiated but still highly organized protoplasm, that is not how life evolved. For about one-half of the 3.6 billion years or so that life has existed on this planet, all organisms were single-celled – first as simple prokaryotic Protista, which apparently combined symbiotically to produce the familiar eukaryotic cell.3 The so-called ‘higher’ animals – including the humans who are the focus of this book – are not made of ever-bigger cells but are instead coordinated aggregates of these tiny droplet complexes of integrated biochemistry. In our case, on the order of 1013 or 1014 (10–100 trillion) of these buzzing little cells somehow work together (coupled with a similar or greater number of enteric bacteria) to produce the event we know as ourselves. We can recognize bundles of these cells even after years of not seeing them or from several blocks away by observing their characteristic manner of movement. What holds our ever-changing soup of cells in such a consistent physical shape?
As in human society, cells within a multicellular organism combine individual autonomy with social interaction. In our own tissues, we can identify four basic classes of cells: neural, muscular, epithelial, and connective tissue cells (each with multiple subtypes) (Fig. 1.2). We could oversimplify the situation a bit by saying that each of these has emphasized one of the functions shared by all cells in general (and the fertilized ovum and stem cells in particular). For instance, all cells conduct along their membranes, but nerve cells have become excellent at it (at a cost, incidentally, to their ability to contract or reproduce well). All cells contain at least some actin, and are thus capable of contraction, but muscle cells have become masters of the art. Epithelial cells also contract, but very feebly, while they specialize in lining surfaces and in the absorption of nutrition and the secretion of chemical products such as hormones, enzymes, and other messenger molecules.
Fig. 1.2 Each of the body’s major cell types specializes in one of the functions shared by the original ovum and the stem cells, e.g. secretion, conduction, contraction, or support. The specialized cells combine into tissues, organs, organisms, and societies.
The fourth type, connective tissue cells are generally less effective at contraction (with one major exception explained later in this chapter) and fairly good as ionic conductors, but their specialty is to secrete an amazing variety of products into the intercellular space that combine to form our bones, cartilage, ligaments, tendons, joints, and fascial sheets. In other words, it is these cells that create the structural substrate for all the others, building the strong, pliable ‘stuff’ that holds us together. This material becomes the shared and communicative environment for all our cells – what Varela4 termed a form of ‘exo-symbiosis’ – shaping us and allowing us directed movement. (As an aside, we cannot let the word ‘environment’ enter our discussion without quoting from the master of the term, Marshall McLuhan:5 ‘Environments are not passive wrappings, but are, rather, active processes which are invisible. The ground-rules, pervasive structure, and overall patterns of environments elude easy perception.’ This may go some way toward explaining why the cellular environment of the extracellular matrix has remained essentially ‘unseen’ for some centuries of research.)
We will leave the discussion of the defensive support offered by the connective tissue cells to the immunologists. We will touch on the trophic and morphogenetic role of connective tissues when we take up embryology and tensegrity later in this chapter.7–9 For now, we concern ourselves with the mechanical support role the connective tissue cells and products offer the body in general and the locomotor system in particular.
The extracellular matrix
The term extracellular matrix (ECM) is applied to the sum total of extracellular substance within the connective tissue. Essentially it consists of a system of insoluble protein fibrils and soluble complexes composed of carbohydrate polymers linked to protein molecules (i.e. they are proteoglycans) which bind water. Mechanically, the ECM has evolved to distribute the stresses of movement and gravity while at the same time maintaining the shape of the different components of the body. It also provides the physico-chemical environment of the cells imbedded in it, forming a framework to which they adhere and on which they can move, maintaining an appropriate porous, hydrated, ionic milieu, through which metabolites and nutrients can diffuse freely.10
This statement is rich, if a little dense; the rest of this chapter is an expansion on these few sentences, pictured in Figure 1.3.
Fig. 1.3 All the connective tissues involve varying concentrations of cells, fibers, and interfibrillar ground substance (proteoaminoglycans). (Reproduced with kind permission from Williams 1995.)
Dr James Oschman refers to the ECM as the living matrix, pointing out that ‘the living matrix is a continuous and dynamic “supermolecular” webwork extending into every nook and cranny of the body: a nuclear matrix within a cellular matrix within a connective tissue matrix. In essence, when you touch a human body, you are touching an intimately connected system composed of virtually all the molecules within the body linked together.’11
Taken altogether, the connective tissue cells and their products act as a continuum, as our ‘organ of form’.12 Our science has spent more time on the molecular interactions that comprise our function while being less thorough on how we shape ourselves, move through environments, and absorb and distribute impact in all its forms – endogenous and exogenous. Our shape is said to be adequately described by our current understanding of anatomy, but how we think about shape results partly from the tools available to us. For the early anatomists, this was principally the knife. ‘Anatomy’ is, after all, separating the parts with a blade. From Galen through Vesalius and beyond, it was the tools of hunting and butchery which were applied to the body, and presented to us the fundamental distinctions we now take for granted (Fig. 1.4). These knives (later scalpels, and then lasers) quite naturally cut along the often bilaminar connective tissue barriers between different tissues, emphasizing the logical distinctions within the extracellular matrix, but obscuring the role of the connective tissue syncytium considered as a whole (Figs 1.5, 7.15 and 7.29).
Fig. 1.4 Vesalius, like other early anatomists given the opportunity to study the human body, exposed the structures with a knife. This legacy of thinking into the body with a blade is with us still, affecting our thinking about what happens inside ourselves. ‘A muscle’ is a concept that proceeds not from recorded physiology but directly from the scalpel approach to the body. (Reproduced with permission from Saunders JB, O’Malley C. Dover Publications; 1973.)
Fig. 1.5 The tensile part of mechanical forces is transmitted by the connective tissues, which are all connected to each other. The joint capsule (1) is continuous with the muscle attachment (2) is continuous with the epimysial fascia (3) is continuous with the tendon (4) is continuous with the periosteum (5) is continuous with the joint capsule (6), etc. For dissections of such continuities in the arm, see Figures 7.7 and 7.29.
If we imagine that instead of using a sharp edge we immersed an animal or a cadaver in some form of detergent or solvent which would wash away all the cellular material and leave only the connective tissue fabric (ECM), we would see the entire continuum, from the basal layer of the skin, through the fibrous cloth surrounding and investing the muscles and organs, and the leathery scaffolding for cartilage and bones (Fig. 1.6A and B). This would be very valuable in showing us this fascial organ as a continuum, emphasizing its uniting, shaping nature rather than simply seeing it as the line where separations are made (Fig. 1.7). This book proceeds from this idea and this chapter attempts to fill in such a picture.
Fig. 1.6 A section of the thigh, derived from the National Library of Medicine’s Visible Human Project by Jeffrey Linn. The more familiar view in (A) includes muscle and epimysial fascia (but not the fat and areolar layers shown in Fig. 1.24). The view in (B) gives us the first glimpse into what the fascial system would look like if that system alone were abstracted from the body as a whole. (Reproduced with kind permission from Jeffrey Linn.)
Fig. 1.7 The fascial matrix of the lower leg (of a rat), showing the histological continuity among synergistic and even antagonistic muscles. This 3-D reconstruction, using three frozen sections of the anterior and lateral crural compartments, enhances the connective tissue structures within each section. The smallest divisions are the endomysial fibers which surround each muscle fiber. The ‘divisions’ between these muscles – so sharp in our anatomy texts – are only barely discernable. (Used with kind permission from Prof. Peter Huijing, PhD, Faculteit Bewegingswetenschappen, Vrije Universiteit Amsterdam.)
We will refer to this body-wide complex as the fascia, or the fascial net. In medicine, the word ‘fascia’ is usually applied more narrowly to the large sheets and woven fabric that invest or surround individual muscles, but we choose to apply it more generally. All naming of parts of the body imposes an artificial, human-perceived distinction on an event that is unitary. Since we are at pains in this book to keep our vision on the whole, undivided, ubiquitous nature of this net, we choose to call it the fascial net. (If you wish, substitute ‘collagenous network’ or ‘connective tissue webbing’ or ‘extracellular matrix’ as decribed in Gray’s Anatomy; here we will go with the simple ‘fascia’.13)
Connective tissue is very aptly named. Although its walls of fabric do act to direct fluids, and create discrete pockets and tubes, its uniting functions far outweigh its separating ones. It binds every cell in the body to its neighbors and even connects, as we shall see, the inner network of each cell to the mechanical state of the entire body. Physiologically, according to Snyder,14 it also ‘connects the numerous branches of medicine’.
Part of its connecting nature may lie in its ability to store and communicate information across the entire body. Each change in pressure (and accompanying tension) on the ECM causes the liquid crystal semiconducting lattice of the wet collagen and other proteins to generate bioelectric signals that precisely mirror the original mechanical information.15 The perineural system, according to Becker, is an ancient and important parallel to the more modern conduction along nerve membranes.16
Although there are a number of different cells within the connective tissue system – red blood cells, white blood cells, fibroblasts, mast cells, glial cells, pigment cells, fat cells, and osteocytes among others – it is the fibroblasts and their close relatives that produce most of the fibrous and interfibrillar elements of such startling and utilitarian variety. It is to the nature of these intercellular elements that we now turn our attention.
The dramatis personae of the connective tissue elements is a short list, given that we are not going to explore the chemistry of its many minor variations. There are three basic types of fibers: collagen, elastin, and reticulin (Fig. 1.8). Reticulin is a very fine fiber, a kind of immature collagen that predominates in the embryo but is largely replaced by collagen in the adult. Elastin, as its name implies, is employed in areas such as the ear, skin, or particular ligaments where elasticity is required. (These elastic fibers may be better classified as another form of collagen.17) Collagen, by far the most common protein in the body, predominates in the fascial net, and is readily seen – indeed, unavoidable – in any dissection or even any cut of meat. There are currently 28 types of collagen fiber, but the distinctions need not concern us here, and Type 1 is by far the most ubiquitous in the structures under discussion. These fibers are composed of amino acids that are assembled like Lego® in the endoplasmic reticulum and Golgi complex of the fibroblast and then extruded into the intercellular space, where they spontaneously (under conditions described below) form into a variety of arrays. That the transparent cornea of the eye, the valves of the heart, the strong tendons of the foot, the spongy lung, and the delicate membranes surrounding the brain are all made out of collagen speaks to its versatility.
Fig. 1.8 This photomicrograph shows very clearly the (purple) fibroblasts in the top third extruding tropocollagen, which combines into the three-strand collagen molecule, apparent in the lower third. There are also bendy yellow elastin fibers, and the much smaller reticulin fibers in the middle third. (© Prof. P. Motta/Science Photo Library. Reproduced with kind permission.)
How to build a body
The list could go on, but the point is made: connective tissue cells make biological correlates of all these materials and more, by playing creatively with cell function and the two elements of the ECM – the tough fiber matrix and the viscous ground substance. The fibers and ground substance, as we shall see, actually form a continuous spectrum of building materials, but the distinction between the two (non-water-soluble collagen fiber and hydrophilic proteoglycans) is commonly used. The ECM, as we will learn in the section on tensegrity, is actually continuous with the intracellular matrix as well, but for now, once again the distinction between what is outside the cell and what is inside is useful.18
Table 1.1 summarizes the way in which the cells alter the fibers and the interfibrillar elements of connective tissue to form all the building materials necessary to our structure and movement.
Table 1.1
To make the situation more complex (as it always is), the ratio between the fibrous element and the calcium salts changes over the course of your life. In a child, the proportion of collagen is higher, so that long bones will break less frequently, having more tensile resilience.17 When they do break, they will often break like a green twig in spring (Fig. 1.9A), fracturing on the side that is put into tension, and rucking up like a carpet on the side that goes into compression. Young bones are difficult to break, but also hard to set back together properly, though they often mend quickly enough due to the responsiveness of the young system and the prevalence of collagen to reknit.
Fig. 1.9 (A) Young bone, with a higher fiber content, breaks like green wood, compressing on one side, splintering on the other. (B) Old bone, with a proportionally higher calcium apatite content, breaks cleanly like dry wood. (Reproduced with kind permission from Dandy 1998.)
In an older person, by contrast, where the collagen is frayed and deteriorated, and thus the proportion of mineral salts is higher, the bone is likely to break like an old twig at the bottom of a pine tree (Fig. 1.9B): straight through the bone in a clean fracture. Easily put back in place but hard to heal, precisely because it is the network of collagen that must cross the break and reknit to itself first, to provide a fibrous scaffolding for the calcium salts to bridge the gap and recreate solid compressional support. For this reason, bone breaks in older people are often pinned to provide solid contact between the surfaces for the extra time required for the remaining collagenous net to link up across the fracture.
Likewise, the various types of cartilage merely reflect different proportions of the elements within it. Hyaline cartilage – as in your nose – represents the standard distribution between collagen and the silicon-like chondroitin sulfate. Elastic cartilage – as in your ear – contains more of the yellowish elastin fibers within the chondroitin. Fibrocartilage – as in the pubic symphysis or intervertebral discs – has a higher proportion of tough fibrous collagen compared to the amount of silicon-like chondroitin.19 In this way, we can see that bone and cartilage are really dense forms of fascial tissue – a difference in degree, rather than a true difference in type.
Connective tissue plasticity
The mechanism of connective tissue remodeling is important to understand if we intend to intervene in human structure and movement. To continue the metaphor for a moment, the human body is a talented ‘building’ that is readily moveable, self-repairs if it is damaged, and actually reconstructs itself over both the short and medium term to respond to different ‘weather conditions’ such as a prevailing wind, a typhoon, or an extended drought.
Stress passing through a material deforms the material, even if only slightly, thereby ‘stretching’ the bonds between the molecules. In biological materials, among others, this creates a slight electric flow through the material known as a piezo- (pressure) electric charge (Fig. 1.10A and B).20 This charge, representative of strain through the tissue, can be ‘read’ by the cells in the vicinity of the charge, and the connective tissue cells are capable of responding by augmenting, reducing, or changing the intercellular elements in the area.
Fig. 1.10 ‘Virtually all the tissues of the body generate electrical fields when they are compressed or stretched [which are] representative of the forces acting on the tissues involved … containing information on the precise nature of the movements taking place … One of the roles of this information is in the control of form’ (Oschman 2000, p. 52). (A) Stress lines in a loaded plastic model of the femur. (From von Knieff 1977.21 Reproduced with kind permission from Williams 1995.) (B) Any mechanical force which creates structural deformation creates such a piezo-electric effect, which then distributes itself around the connective tissue system. (Reproduced with kind permission from Oschman 2000.) (C) The trabeculae of bone which form in response to individualized stresses. (Reproduced with kind permission from Williams 1995.)
As an example, the head of most everyone’s femur is made of cancellous, spongy bone. An analysis of the trabeculae within the bone shows that they are brilliantly constructed, to an engineer’s eye, to resist the forces being transmitted from the pelvis to the shaft of the femur. Such an arrangement provides us the lightest bones possible within the parameters of safety, and could easily be explained by the action of natural selection. But the situation is more complex than that; the internal bone is shaped to reflect not only species’ needs but also individual form and activity. If we were to section the femur of someone with one posture and someone else with a quite different posture and usage, we would see that each femoral head has slightly different trabeculae, precisely designed to best resist the forces which that particular person characteristically creates (Fig. 1.10C). In this way, the connective tissue responds to demand. Whatever demand you put on the body – continuous exertion or dedicated couch potato, running 50 miles a week or squatting 50 hours a week in the rice paddies – the extracellular elements are altered along the path of the stress to meet the demand within the limits imposed by nutrition, age, and protein synthesis (genetics).
In bone the currents of stress perform this seeming miracle of preferential remodeling within the intercellular elements via a sparse but active community of two types of osteocytes: the osteoblasts and the osteoclasts. Each are sent forth with simple commandments: osteoblasts lay down new bone; osteoclasts clean up old bone. Osteoblasts are allowed to lay down new bone anywhere they like – as long as it is within the periosteum. The osteoclasts may eat of any bone, except those parts that are piezo-electrically charged (mechanically stressed).22 Allow the cells to operate freely under these rules over time, and a femoral head is produced that is both specifically designed to resist individual forces coming through it, but also capable of changing (given some reaction time) to meet new forces when they are consistently applied.
This extraordinary ability to respond to demand accounts for the wide variety in joint shapes across the human spectrum, despite the consistent pictures averaged into most anatomy textbooks. A recent study detailed distinct differences in the structure of the subtalar joint.23 Smaller differences can be observed over the entire body. In Figure 1.11A we see a ‘normal’ thoracic vertebra. However, in Figure 1.11B, we can see the body distorted as pressure creates a demand for remodeling under Wolff’s law,24 and hypertrophic spurs forming as the periosteum is pulled away by excess strains from the surrounding connective tissues and muscles (see also Ch. 3 on heel spurs). A non-union fracture can often be reversed by creating a current flow across the break, reproducing the normal piezo-electric flow, through which the collagen orients itself and begins the process of bridging the gap, to be followed by the calcium salts and full healing.25,26
Fig. 1.11 Even bones will alter their shape within certain limits, adding and subtracting bone mass, in response to the mechanical forces around them. (Reproduced with kind permission from Oschman 2000.)
This same process of response occurs across the entire extracellular fibrous network, not just inside the bones. We can imagine a person who develops for whatever reason (e.g. shortsightedness, depression, imitation, or injury) a common ‘slump’: the head goes forward, the chest falls, the back rounds (Fig. 1.12). The head, a minimum of one-seventh of the body weight in most adults, must be restrained from falling further forward by some muscles in the back. These muscles must remain in isometric/eccentric contraction (eccentric loading) for every one of this person’s waking hours.
Fig. 1.12 When body segments are pulled out of place and muscles are required to maintain static positions – either stretched/contracted (‘locked long’) or shortened/contracted (‘locked short’) – then we see increased fascial bonding and thixotropy of the surrounding intercellular matrix (ECM).
Muscles are designed to contract and relax in succession, but these particular muscles are now under a constant strain, a strain that robs them of their full ability, and facilitates the development of trigger points. The strain transmits through the fascia within and around the muscle (and often beyond in both directions along the myofascial meridians). Essentially, these muscles or parts of muscles are being asked to act like straps (Fig. 1.13A and B).
Fig. 1.13 (A) The ECM is designed to allow the relatively free flow of metabolites from blood to cell and back again in the flow of interstitial fluid and lymph. (B) Chronic mechanical stress through an area results in increased laying down of collagen fiber and decreased hydration of the ECM’s ground substance, both of which result in decreased nourishment to certain cells in the ‘back-eddies’ caused by the increased matrix.
Stretched, a muscle will attempt to recoil back to its resting length before giving up and adding more cells and sarcomeres to bridge the gap.27 Stretch fascia quickly and it will tear (the most frequent form of connective tissue injury). If the stretch is applied slowly enough, it will deform plastically: it will change its length and retain that change. Slowly stretch a common plastic carrier bag to see this kind of plasticity modeled: the bag will stretch, and when you let go, the stretched area will remain, and will not recoil.
In short, muscle is elastic, fascia is plastic.28,29 While this is a clinically useful generalization for the manual therapist, it is definitively not true. We have already mentioned that some fascial tissues – the ear, for instance – have higher proportions of elastin which renders the non-muscular tissue quite deformably elastic over a fairly large range. Beyond that, however, arrangements of pure collagen such as tendons, ligaments, and aponeuroses have tighter elastic properties that allow for the brief storage of significant energy in extension and a recoil shortening as that energy is ‘given back’. The Achilles tendon, for instance, is quite compliant, and it has been shown that in human walking and running the triceps surae (soleus and gastrocnemii) basically contract isometrically while the tendon cycles through stretching and shortening.30–33
The mechanism of fascial plastic deformation (viscoelasticity as opposed to elasticity) is incompletely understood, but once it is truly deformed, fascia does not ‘snap back’. Over time and given the opportunity – i.e. bringing the two fascial surfaces into apposition again and keeping them there, as in going back into the slump – it will, however, lay down new fibers that will rebind the area.34 But this is not the same as elastic recoil in the tissue itself. A full understanding of this concept is fundamental to the successful application of sequential fascial manipulation. Practicing therapists, in our experience, make frequent statements that betray an underlying belief that the fascia is either elastic or voluntarily contractile, even though they ‘know’ it is not. The plasticity of fascia is its essential nature – its gift to the body and the key to unraveling its long-term patterns. We will return to fascial contractility and elasticity at the cellular level in the section on ‘tensegrity’ below.
Back to our slump: eventually, fibroblasts in the area (and additional mesenchymal stem cells or fibroblasts that may migrate there) secrete more collagen in and around the muscle to create a better strap. The long collagen molecules, secreted into the intercellular space by the fibroblasts, are polarized and orient themselves like compass needles along the line of mechanical tension (Fig. 1.14). They bind to each other with numerous hydrogen bonds via the interfibrillar glue (proteoglycans or ground substance), forming a strap-like matrix around the muscle.
Fig. 1.14 (A) The collagen molecules, manufactured in the fibroblast and secreted into the intercellular space, are polarized so that they orient themselves along the line of tension and create a strap to resist that tension. In a tendon, almost all fibers line up in rows like soldiers. (Reproduced with kind permission from Juhan 1987.79) (B) If there is no ‘prevailing tension’, the collagen fibers (CF) will orient willy-nilly, as in felt. (Reproduced from Kessel and Kardon 1979.35)
Figure 1.15 illustrates this phenomenon very well. It shows a dissection of some of the fascial fibers running over the sternum between the two pectoral muscles. If we compare the fibers running from upper right to lower left, we can see that they are denser and stronger than those running from the upper left to the lower right. This means that more strain was habitually present in that one direction, perhaps from being left-handed, or (entirely speculatively) from being a big city bus driver who used his left hand predominantly to swing his horizontal steering wheel. This strain caused lines of piezo-electricity, and the fibroblasts responded by laying down new collagen, which oriented along the lines of strain to create more resistance.
Fig. 1.15 A dissection of the superficial pectoral fascia in the sternal area. Notice how one leg of the evident ‘X’ across the sternum, from upper right to lower left in the picture, is more prevalent than the other, almost certainly as a result of use patterns. (Reproduced with kind permission from Ronald Thompson.)
1. a reopening of the tissue in question, to help restore fluid flow, muscle function, and connection with the sensory–motor system, and
2. an easing of the biomechanical pull that caused the increased stress on that tissue in the first place.
Either of these alone produces temporary or unsatisfactory results. The second point urges us to look beyond ‘chasing the pain’ and calls to mind the prominent physiotherapist Diane Lee’s admonition: ‘It is the victims who cry out, not the criminals.’ Taking care of the victims and collaring the local thugs is addressed by point 1, going after the ‘big shots’ is the job of point 2.
In the slump pictured in Figure 1.12 (reminiscent of Vladimir Janda’s upper crossed syndrome36), the muscles in the back of the neck and top of the shoulders will have become tense, fibrotic, and strained, and will require some work. But the concentric pull in the front, be it from the chest, belly, hips, or elsewhere, will need lengthening first, and the structures beneath it rearranged to support the body in its ‘new’ (or more often ‘original’, natural) position.
In other words, we must look globally, act locally, and then act globally to integrate our local remedies into the whole person’s structure. In strategizing our therapy in this global–local–global way, we are acting exactly as the ECM itself does, as we will explore below in the section on tensegrity. Connective tissue cells produce ECM in response to local conditions, which in turn affect global conditions that re-impinge on local conditions in an unending recursive process.37 Understanding of the myofascial meridians assists in organizing the search for both the silent culprit and the necessary global decompensations – reversing the downward spiral of increasing immobility.
More serious deformations of the fascial net may require more time, remedial exercise, peri-articular manipulation (such as is found in osteopathy and chiropractic), outside support such as orthotics or braces, or even surgical intervention, but the process described above is continual and ubiquitous. Much restoration of postural balance, whether via the Anatomy Trains scheme or any other model, is attainable using non-invasive techniques. A preventive program of structural awareness (call it ‘kinesthetic literacy’) could also be fairly easily and productively incorporated into public education.38–41
These metaphors are presented in brief and general terms – there is no space to flesh them out fully and still attend to our primary purpose. For the more scientifically minded, we note that aspects of these metaphors run ahead of the supporting research. Nevertheless, some speculative exploration seems useful at this point. Anatomy has been thoroughly explored in the previous 450 years. New discoveries and new therapeutic strategies will not come from finding new structures, but from looking at the known structures in new ways.
The three holistic networks
The neural net
If we could make everything invisible around it and leave the nervous system standing as if in life (a tall order even for magic, considering the nervous system’s fragility), we would see the exact shape of the body, entirely and with all the individual variations (Fig. 1.16). We would see the brain, of course, which Vesalius unaccountably omitted, and the spinal cord, which he left encased in the vertebrae. All the main trunks of the spinal and cranial nerves would branch out into smaller and smaller twigs until we reached the tiny tendrils which insinuate themselves into every part of the skin, locomotor system, and organs. Vesalius presents only the major trunks of nerves, the smaller ones being too delicate for his methods. A more modern and detailed version, albeit still with only the major nerve trunks represented, can be seen in the Sacred Mirrors artwork at www.alexgrey.com.
Fig. 1.16 It is amazing, given the methods available at the time, that Vesalius could make such an accurate version of the delicate nervous system. A modern and strictly accurate version of just this system would not include the spine, as Vesalius did, and would, of course, additionally include the brain, the autonomic nerves, and the many finer fibers he was unable to dissect out. (Reproduced with permission from Saunders JB, O’Malley C. Dover Publications; 1973.)
We would clearly see each organ of the ventral cavity in the filmy autonomic system reaching out from the sympathetic and parasympathetic trunks. The digestive system is surrounded by the submucosal plexus, which has as many neurons spread along the nine yards of the digestive system as are in the brain.42 The heart would be particularly vivid with the bundles and nodes of nerves that keep it tuned.
Of course, this system is not equally distributed throughout; the tongue and lips are more densely innervated than the back of the leg by a factor of 10 or more. The more sensitive parts (e.g. the hands, the face, the genitals, the eye and neck muscles) would show up with greater density in our filmy ‘neural person’, while the otherwise dense tissues of bones and cartilage would be more sparsely represented. No part of the body, however, save the open lumens of the circulatory, respiratory, and digestive tubes, would be left out.
The fluid net
Similarly, if we made everything invisible but the vascular system, we would once again have a filmy representation that would show us the exact shape of the body in question (Fig. 1.17). Centered around the heart’s incessant pump, its major arteries and veins go to and from the lungs, and out through the aorta and arteries to the organs and every part of the body via the wide network of capillaries.
Fig. 1.17 Vesalius, in 1548, also created a picture of our second whole-body system, the circulatory system. (Reproduced with permission from Saunders JB, O’Malley C. Dover Publications; 1973.)
Although the concept can clearly be seen in the early attempt by Vesalius, notice that in his conception the veins and arteries do not join with each other – it would take another two centuries for William Harvey to discover capillaries and the closed nature of the circulatory net. A full accounting would show tens of thousands of miles (about 100 000 km) of capillary nets, giving us another filmy ‘vascular body’ that would be complete down to the finest detail (Figs 1.18–1.20 or see the complete system modeled at www.bodyworlds.com). If we included the lymphatic and the cerebrospinal fluid circulation in our consideration of the vascular system, our ‘fluid human’ would be even more complete, down to the finest nuances of everything except hair and some gaps created by the avascular parts of cartilage and dense bone.
Fig. 1.18 A cast of the venous system inside the liver from below. The sac in the center is the gall bladder. (© Ralph T. Hutchings. Reproduced from Abrahams, et al. 1998.)
Fig. 1.19 Even with just these few large arteries represented, we can see something about this person. You might guess a Nilo-Hamitic person, for instance, but it is, in fact, a full-term infant. (© Ralph T. Hutchings. Reproduced from Abrahams, et al. 1998.)
Fig. 1.20 Even the brain itself is full of blood vessels (and the heart is full of nerves). Is it only the neurons of the brain that ‘think’? (© Ralph T. Hutchings. Reproduced from Abrahams, et al 1998.)
In any multicellular organism – and especially true for those who have crawled out onto dry land – the inner cells, which are not in direct communication with the outside world, depend on the vascular system to bring nourishing chemistry from the outer edges of the organism to the middle, and to take otherwise toxic chemistry from the middle to the edge where it can be dispersed. The organs of the ventral cavity – the lungs, the heart, the digestive system, and the kidneys – are designed to provide this service for the inner cells of the body. To provide a comprehensive ‘inner sea’ complete with nourishing and cleansing currents, the network of capillaries must penetrate into the immediate neighborhood of most individual cells, of whatever type, to be able to deliver the goods via diffusion from the capillary walls. Cartilage and ligament injuries take longer to heal because their cells are so far from the shores of this inner sea that they must rely on seepage from farther away.
The fibrous net
It can be no surprise, given our subject, that the fascial system is our third whole-body communicating network; the only surprise is how little the importance of this network has been recognized and studied as a whole until recently (Fig. 1.21).
Fig. 1.21 (A) Vesalius shows the fibrous net in the familiar way – as a layer of muscles – but the overlying layers of fascial fabric have been removed. (B) The second view shows a deeper layer of musculature; fascial septa would fill in all the gaps and lines among the muscles. In (B), notice the black line extending from the bottom of the diaphragm to the inside arch of the foot, and compare it to the Deep Front Line (see Ch. 9). (Reproduced with permission from Saunders JB, O’Malley C. Dover Publications; 1973.)
If we were to render all tissues invisible in the human body except the fibrillar elements of the connective tissue – collagen, elastin and reticulin – we would see the entire body, inside and out, in a fashion similar to the neural and vascular nets, though the areas of density would once again differ. The bones, cartilage, tendons, and ligaments would be thick with leathery fiber, so that the area around each joint would be especially well represented. Each muscle would be sheathed with it, and infused with a cotton-candy net surrounding each muscle cell and bundle of cells (see Fig. 1.1B). The face would be less dense, as would the more spongy organs like the spleen or pancreas, though even these are encased by denser, tough bags. Although it arranges itself in multiple folded planes, we emphasize once again that no part of this net would be distinct or separated from the net as a whole; each of these bags, strings, sheets, and leathery networks is linked to each other, top to toe. The center of this network would be our mechanical center of gravity, located in the middle of the lower belly in the standing body, known in martial arts as the ‘hara’.
This ubiquitous network has enough of a regular molecular lattice (see Fig. 1.14A) to qualify as a liquid crystal, which begs us to question to what frequencies this biological ‘antenna’ is tuned, and how it can be tuned to a wider spectrum of frequencies or harmonized within itself. Although this idea may seem farfetched, the electrical properties of fascia have been noted but little studied to date, and we are now glimpsing some of the mechanisms of such ‘tuning’ (pre-stress – see the section on tensegrity below).43–46
In contrast to the neural and vascular net, the fascial net has yet to be depicted on its own by any artist we have seen to date. Vesalius’s closest rendering is the familiar écorché view of the body (Fig. 1.21), which certainly gives us some idea of the grain of the fabric of the fibrous body, but really renders the myofascia – muscle and fascia together, with a heavy emphasis on the muscle. This is a prejudgment that has been continued in many anatomies, including those in wide use today: the fascia is largely removed and discarded to give visual access to the muscles and other underlying tissues.45–49
These common depictions have also removed and discarded two important superficial fascial layers: the dermis that provides a carpet backing for the skin, and the fatty areolar layer with its well-funded store of white blood cells (Fig. 1.22). If we left these hefty layers in the full picture, we would see the animal equivalent of a citrus ‘rind’ beneath the very thin skin. Removing these layers and the rest of the ‘packing material’ helps contribute to a view of the fascial net as a ‘dead’ scaffolding around the cells, to be parted and discarded on the way to the ‘good stuff’. Now, however, we are at pains to reverse this trend to create a picture of the fascial net with everything else, including the muscle fibers, removed.
Fig. 1.22 (A) An extraordinary one-piece dissection of the areolar/adipose layer of superficial fascia fills in the picture not covered by Figure 1.21 (or Fig. 1.6). This picture does not include the dermis layer of the skin, but does include the fat, the collagen matrix around the fat, and of course the many leucocytes at the histological level. (B) Here we see the specimen in full along with the donor who provided it. Hedley’s concept of this fascial layer as a nearly autonomous organ, somewhat akin to the rind of the grapefruit pictured in Figure 1.23, is given a concrete reality through this feat of dissection. (© Gil Hedley 2005. www.gilhedley.com. Used with kind permission.)
New methods of depicting anatomy have brought us very close to this picture. Structural Integration practitioner Jeffrey Linn,50 using the Visible Human Project data set, created Figure 1.1C by mathematically eliminating everything that was not fascia in a section of the thigh; he gives us the closest approximation of a ‘fascial human’ we yet have – though this view also omits the two superficial fascial layers.
It will be some time before such methods can be used to show the entire fascial system, for it would include (as Fig. 1.1C does not, but Fig. 1.1B does) the cotton wool infusing each and every muscle, as well as the perineural system of oligodendrocytes, Schwann cells, and glial cells and attendant fats which cuddle the nervous system, as well as the complex of bags, ligaments, and spider webs that contain, fix, and organize the ventral organ systems.
A grapefruit provides a good metaphor for what we are trying to envision (Fig. 1.23). Imagine that you could somehow magically extract all the juice out of a grapefruit without disturbing the structure within. You would still have the shape of the grapefruit intact with the rind of the dermis and areolar layers, and you would see all the supporting walls of the sections (which, if dissected would turn out to be double-walled membranes, one half going with each section – just like our intermuscular septa). Plus we would see all the little filmy walls that separated the single cells of juice within each section. The fascial net provides the same service in us, except it is constructed out of pliable collagen instead of the more rigid cellulose. The fascial bags organize our ‘juice’ into discrete bundles, resisting the call of gravity to pool at the bottom. This role of directing and organizing fluids within the body is primary to an understanding of how manual or kinetic therapy of this matrix can affect health.
Fig. 1.23 A person is not unlike a grapefruit in construction. The skin is much like our own skin – designed to deal with the outside world. The rind is akin to the ‘fat suit’ we all wear, seen in Figure 1.22. Each segment is separated from the next by a wall we see when we cut the grapefruit through the equator for breakfast. But if we peel it and separate the sections as we might with an orange, we realize that the seeming one wall is actually two walls – one half goes with each section. The intermuscular septa are the same way. We often separate them with a knife, so we think of them as simply the epimysium of each muscle. But just as the walls are left after we eat a grapefruit, the walls are what is left in Figure 1.6, and we can see what strong structures they are, worthy of separate consideration.
It is worth our while to focus our microscope in for a moment, to see this sugary glue in action.
In Figure 1.13, we imagine ourselves at the cellular level (similar to Fig. 1.3). The cells are deliberately left blank and undefined; they could be any cells – liver cells, brain cells, muscle cells. Nearby is a capillary; when the blood is pushed into the capillary by systole of the heart, its walls expand and some of the blood is forced – the plasma part, for the red blood cells are too stiff to make it through – into the interstitial space. This fluid carries with it the oxygen, nutrients, and chemical messengers carried by the blood, all intended for these cells. In between lies the stuff that occupies the intercellular realm: the fibers of the connective tissue, the interfibrillar mucousy ground substance, and the interstitial fluid itself, which is very similar (indeed, readily interchangeable) to the blood’s plasma and lymph. The plasma, termed interstitial fluid when it is pushed through the capillary walls, must run the gauntlet of the connective tissue matrix – both fibrous and gluey – to get the nourishment and other messenger molecules into the target cells. The denser the mesh of fiber and the less hydrated the ground substance, the more difficult that job becomes. Cells lost in the ‘back-eddies’ of fluid circulation will not function optimally. (See Fig. 1.3 and the accompanying discussion.)
How easily the nutrients make it to the target cells is determined by:
If the fibers are too dense, or the ground substance too dehydrated and viscous, then these cells will be less thoroughly fed and watered. It is one basic intention of manual and movement interventions – quite aside from the educational value they may have – to open both of these elements to allow free flow of nutrients to, and waste products from, these cells. The condition of the fibers and ground substance is of course partially determined by genetic and nutritional factors, as well as exercise, but local areas can be subject to ‘clogging’ through either fiber or glue when excess strain, trauma, or insufficient movement has allowed such clogging to occur. Once the clog is dispersed, by whatever means, the free flow of chemistry to and from the cells allows the cell to stop functioning on metabolism-only, ‘survival’ mode to resume its specialized ‘social’ function, be that contraction, secretion, or conduction. ‘There is but one disease,’ says Paracelsus,51 ‘and its name is congestion.’
Back at the macro level, we need one final note on the distribution of the net in general: it is worthwhile making a separation, for clinical analysis only, among the fibrous elements inhabiting the two major body cavities – dorsal and ventral (Fig. 1.24).
Fig. 1.24 The subject of this book is the myofascia in the body’s locomotor chassis. But the connective tissue net extends into the dorsal (yellow) and ventral (red) cavities as well, to surround and invest the organs. (Image provided by Dr N. Roberts, Magnetic Resonance Centre, University of Liverpool. Reproduced with kind permission from Williams 1995.)
The dura mater, arachnoid layer, and pia mater are connective tissue sacs that surround and protect the brain, and are in turn surrounded by and awash in the cerebrospinal fluid (CSF). These membranes arise from the neural crest, a special area at the junction between the mesoderm and ectoderm in the developing embryo.52 They interact with the central nervous system and the CSF to produce a series of palpable pulses within the dorsal cavity, and by extension, to the fascial net as a whole.53–55 These pulses are well known to the cranial osteopaths and others who use them therapeutically, though the mechanism is not yet well understood, and even the existence of these wave motions is still denied by some.56,57
Besides the billions of neurons that make up the brain and spinal cord, within the dorsal cavity there are additional connective tissue cells that surround and infuse the entire nervous system, called the perineural network. These astrocytes, oligodendrocytes, Schwann cells, and other neuroglia are ‘greater in number [than the neurons] but have received less attention because they were not thought to be directly involved in neural transmission’, according to Charles Leonard.58 In fact, the neuroglia outnumber the neurons about 10 : 1. Now they are ‘beginning to cast a shadow over the performing brilliance of the neurons’. During development, support cells guide the neurons to their final destination, provide nutrients to neurons, create protective barriers, secrete neuroprotective chemicals, and literally provide the glue and skeleton to hold the nervous system together. Recent research has pointed to the participation of the neuroglia in brain function, particularly in the area of emotional feelings.59 Neuroglia also apparently act as ‘gatekeepers’ to the synapse, sorting which of the neuropeptides are allowed into the synaptic space to affect neural transmission, as well as helping to sweep up excess neurotransmitter in the synaptic cleft.60
The perineural cells also have a signal transmission system of their own, perhaps a more ancient precursor to the highly specific digital capabilities of the neuronal transmission. In normal functioning and in wound healing, the slow waves of DC current that run along the perineural network help to organize generation and regeneration, and may act as a kind of integrating ‘pacemaker’ for the organism.61–63
In embryological development, the perineural cells take on a morphogenetic role. For example, the cells of the neocortex are born deep in the brain on the shores of the ventricles. Yet they must locate themselves incredibly precisely in a layer exactly six cells thick, on the very surface of the brain. These developing neurons use long extensions of neighboring neuroglia, gliding up the extension like a diver using a guide rope, ushered to their precise final position on the brain’s surface by the supporting connective tissue network.64
The temptation to jump the gun and give this perineural network a role in consciousness is barely resistable.65,66
The French physiotherapist and osteopath Jean-Pierre Barral has made an interesting observation that these interfacing surfaces of serous membranes moving on each other could be thought of as a series of inter-organ ‘joints’.67 He has made a fascinating study of the normal excursion of the organs within their fascial bags during breathing, as well as their inherent motility (a motion similar to the craniosacral pulse). According to Barral, the ligaments that attach these organs to surrounding structures determine their normal axes of movement. Any additional minor adhesions that restrict or skew these motions (which are, after all, repeated close to 20 000 times each day) can adversely affect not only organ function over time, but also expand into the surrounding myofascial superstructure.
• The meninges and perineurium that surround and pervade the predominantly ectodermal tissues of the dorsal cavity, currently dealt with by the methods similar to cranial osteopathy, craniosacral therapy, sacro-occipital technique, and methods of dealing with adverse neural tension in the cranial and peripheral nerve sheaths;
• The peritoneal, pleural, and pericardial sacs and their ligamentous attachments that surround and pervade the predominantly endodermal tissues of the ventral cavity are addressed by the techniques and insights of visceral manipulation and Asian organ releases;
• The ‘outer bag’ (see the following section on embryology for an explanation of this term) of myofascia, which contains all of the myofascial meridians described herein and yields to the many forms of soft-tissue bodywork such as strain–counterstrain, trigger-point therapy, myofascial release, and structural integration, and finally
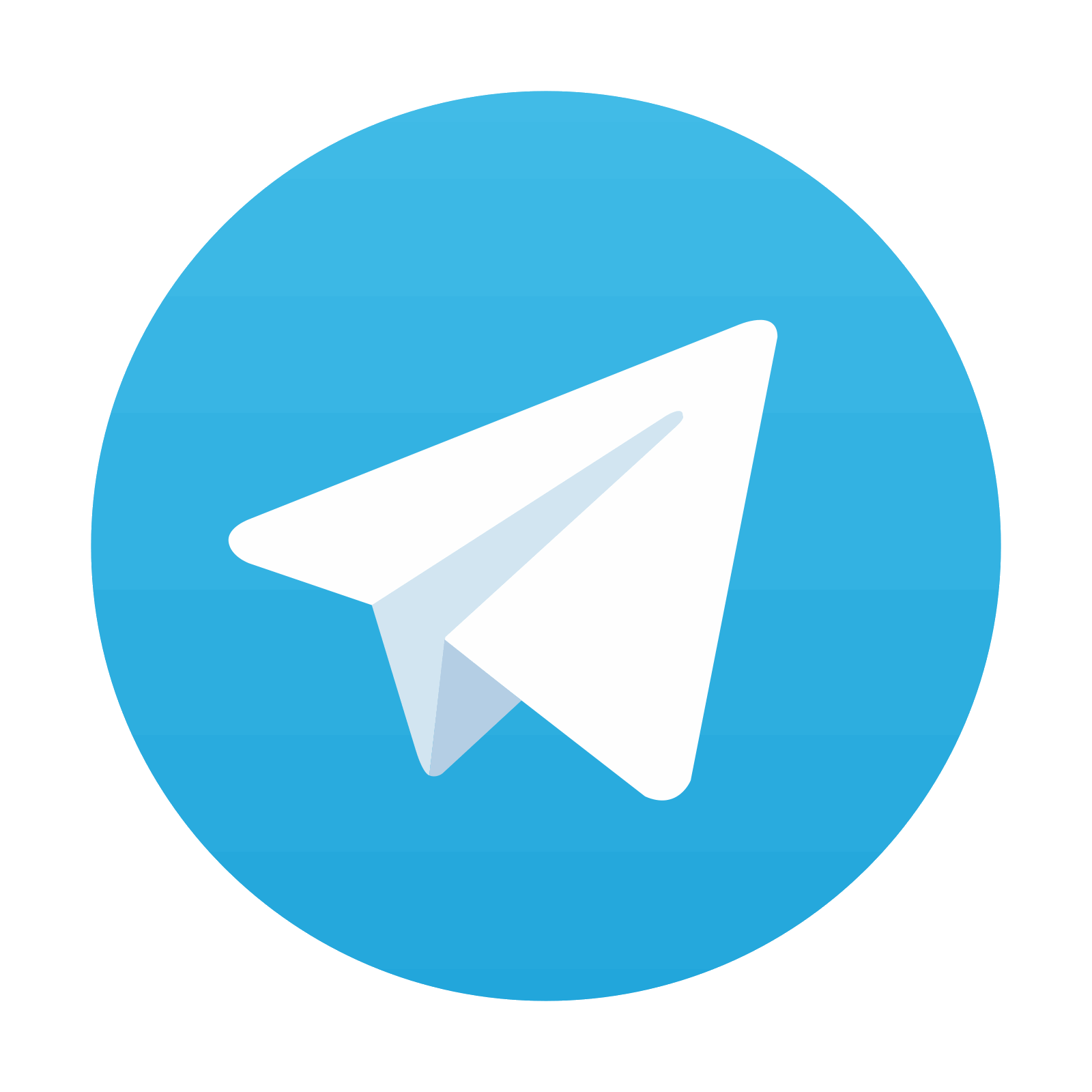
Stay updated, free articles. Join our Telegram channel
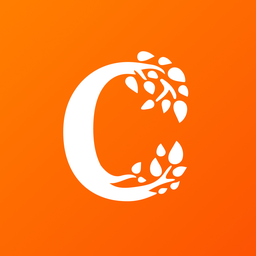
Full access? Get Clinical Tree
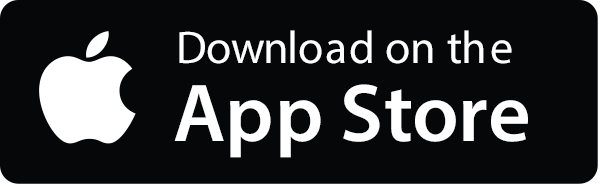
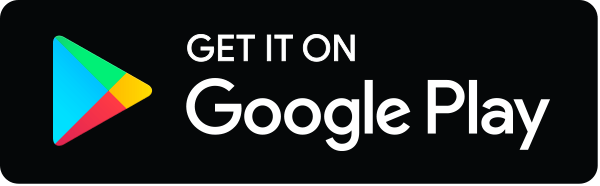