Fluid-Filled Spaces
These sites provide the most readily interpretable data on extravascular drug distribution. The drug accumulates by means of passive diffusion, specimens rarely are contaminated by blood, and repeated samples can be obtained to profile the drug distribution and determine equilibrium conditions. Sites such as ascitic fluid, joint effusions, pleural effusions, amniotic fluid, pericardial effusions, bursae, blisters, and abscesses are classified in this category. These sites are also characterized by having a relatively small surface area for diffusion, compared with their volume. This results in a slow accumulation of antibiotic with repeated dosing, as well as lower peak and higher trough drug concentrations than those of serum (3,4). In humans, the need to perform an invasive procedure (needle aspiration) to obtain fluid limits the utility of these sites for routine sampling, but cutaneous blister models in humans and tissue cages and semipermeable implanted chambers in animals provide useful models of this type of extravascular site (5–7).
Excretory and Secretory Fluids
These fluids provide the greatest variability in achievable drug concentrations of all extravascular sites. Because many antimicrobials are actively secreted into urine or bile, tremendous concentration of drug is possible. In contrast, many glands and organs appear to pose a substantial barrier to penetration by antimicrobials (e.g., milk and prostatic fluid). Numerous glandular secretions have been studied for antimicrobial penetration, but bile, urine, sputum, and milk are the most frequently examined. Many of these fluids are readily obtained without the aid of invasive techniques (urine, sputum, milk, and saliva) and thus have been repetitively sampled over time to garner data on secretory rates. As with fluid-filled spaces, secretory fluids usually are free of blood contamination and thus provide the opportunity to determine antimicrobial concentrations quite reliably.
Spaces with Diffusion Barriers
Two body sites, the eye and the CSF, have traditionally been treated as unique because of the low concentration of many antimicrobials achieved there. Other secretory organs, such as breast and prostate, also pose barriers to drug penetration but are included under secretory organs. Although referred to as barriers to diffusion, active transport from CSF to blood or removal, by bulk CSF flow, of certain compounds such as penicillins may be a major reason for the low concentrations in CSF (8). Again, these sites provide reliable data about drug penetration because of their usual lack of blood contamination. However, the invasive nature of procedures used to obtain these fluids limits the number of determinations that can be made in patients.
Whole-body Tissues
Concentrations of antimicrobials in whole tissues are frequently difficult to interpret because of contamination of the tissues by blood and body fluids. Tissues have distinct compartments including interstitial fluid and cells, and cells contain various subcellular organelles and cytosol. Antimicrobial concentrations vary among these compartments. Some antimicrobials such as quinolones and macrolides attain high intracellular concentrations, whereas β-lactams and aminoglycosides do not. Further, the site of the infection may vary from being primarily extracellular or intracellular, making the knowledge of total tissue concentrations of questionable value (9). Despite these interpretation difficulties, whole tissue samples are among the specimens most frequently obtained for antimicrobial assay. We have included in this chapter our recommendations for the preparation and assay of whole tissues in order to make these determinations more reliable.
MODELS OF EXTRAVASCULAR ANTIBIOTIC DISTRIBUTION IN HUMANS
The use of human experimental models for extravascular distribution of antimicrobial agents is a potentially important development because it allows the controlled investigation of complex pharmacokinetic relationships seen in humans as intact individuals (10). The proper use and interpretation of these models can lead to a clearer understanding of the nature of drug distribution to sites outside the extravascular space. The primary experimental models currently under investigation include so-called skin-window techniques (both disk and chamber), skin-blister models (created by suction and irritant cantharides), the implantation of subcutaneous threads, and the use of microdialysis probes inserted into muscle or adipose tissue. The most useful models are likely to be the skin-blister models and microdialysis probes. The use of skin blisters provide sufficient volume of locally produced extravascular fluid to enable enough samples to be taken both for evaluation of the chemical composition of the extravascular fluid and for multiple antimicrobial concentration determinations so that a complete drug area under the curve (AUC) for extravascular fluid can be measured. This complete evaluation makes comparison to intravascular kinetic data more meaningful and valid conclusions more likely. Similarly, the use of microdialysis probes is a minimally invasive technique that provides complete pharmacokinetic data, including AUC data, of non–protein-bound antimicrobial concentrations within the interstial space of a tissue.
Skin-Window Disk
This technique was described by Raeburn (10) as a modification of the skin-window method of Rebuck and Crowley (11). A 25-mm2 area of skin is abraded using a 25,000-rpm motorized buffer. The abrasion is done (without bleeding) just prior to measurement of the antibiotic. A sterile paper antibiotic disk (6- or 12-mm diameter) is then applied directly to the abraded area and covered with a sterile glass slide, which is secured in position for a selected period of time (usually 1 hour). New applications are made for each additional 1 hour that tissue fluid is to be sampled. Assays are performed by the bioassay technique using paper disks. The amount of tissue fluid on the disk is quantitated by a comparison of disk weight before and after application. Antibiotic standards for the bioassay should contain the same weight of fluid as the unknown specimen (12), a problem of considerable difficulty because disks increase their absorptive capacity with increasing time in contact with fluid, even up to 24 hours (13). The standards should be prepared in a diluent with a protein content similar to that of the tissue fluid (14). The latter point is particularly important for the assay of highly protein-bound drugs and has not been addressed in published studies with this model (10,12). The method allows for measurement of fluid for a relatively short time period at each site and appears to sample tissue fluid that is absorbed from the denuded dermis by the capillary action of the paper disk. However, the limited amount of fluid obtained makes analysis of its composition difficult.
Skin-Window Chamber
This method, described by Tan et al. (15) and used by Tan and Salstrom (16,17), is also a modification of the Rebuck and Crowley (11) skin-window technique. The dermis is exposed by scraping the epidermis with a scalpel blade and placing a tissue culture chamber, with one glass window removed, over the exposed dermis (18). The chamber is taped in place and filled with 1 mL of sterile saline under slightly negative pressure. During study of a drug, the chamber is periodically emptied and refilled (usually at hourly intervals). The method results in marked dilution of the tissue fluid by saline or buffer. The assumption that equilibration of chamber and tissue fluid occurs within a 1-hour period is likely erroneous, based on the limited diffusing surface area compared with chamber volume. Protein content of the saline fluid is low, as anticipated, and therefore, this method is likely to show an apparent reduced penetration of highly bound drugs, compared with systems containing higher levels of albumin (7).
Skin Blister by Suction
This method is based on work by Kiistala and Mustakallio (19), who demonstrated a noninflammatory separation of the epidermis from the dermis by the application of negative pressure. The method used a Perspex block with eight 8-mm holes drilled in it (17). The block is tightly applied to the skin and 0.3 kg/cm2 of pressure is applied to each bore for approximately 2 hours. A block applied to each forearm yields 16 0.15-mL blisters. A different blister is available for evacuation at each sample time. Blisters differ from the skin-window chambers in having fluid with high, rather than low, protein levels. This fluid is produced by the host without the addition of extraneous buffer.
Skin Blister by Cantharides
Cutaneous blisters can also be formed by application of the skin irritant, cantharides, which is prepared from the dried Spanish fly (Cantharis vesicatoria) or blister bug. Cantharidin, the most important active ingredient in Spanish fly, has also been used. Simon and Malerczyk (20) were probably the first to use this method. A plaster of 0.2% cantharides (or cantharidin ointment) is applied to the forearms in a 1-cm2 area 8 to 12 hours before study (21). After formation, the blisters are repeatedly sampled with a fine-bore needle and syringe or capillary tube and the integrity of the blister is maintained by spraying the puncture site with a plastic dressing. The total protein and albumin contents of this inflammatory blister model are the highest of the models currently in use (21).
Subcutaneous Threads
Another method for the study of cutaneous antibiotic penetration in humans (22–24) is the subcutaneous thread. An area of the skin is anesthetized locally with lidocaine and multiple umbilical tapes are threaded under the skin with a needle for a distance of 1 to 2 cm. Studies are begun 30 minutes after placement. Tapes are advanced 1 to 2 cm after each time interval to be measured (for long tapes) or removed completely (when multiple short tapes are implanted), and the subcutaneous segment is cut off and assayed. Assay is typically performed by cutting the tape into 1-cm lengths with different concentrations of antibiotic in each piece. Tapes may also be left in place 24 hours prior to antibiotic administration, but this results in a greater inflammatory response (24). The method allows only a limited time for diffusion of tissue fluid at the site when long tapes are continuously advanced, but this problem can be overcome by the implantation of multiple short lengths of umbilical tape. The umbilical tape has a very large surface area exposed for absorption because of the porous cotton weave of the thread. This model poses similar problems for standardization as seen with the paper disk models (13).
Microdialysis Probes
Muller et al. (25) first described the method of measuring antimicrobial concentrations by use of microdialysis probes. Antimicrobial concentrations within the free interstitial fluid of a tissue are measured by insertion of a microdialysis probe into tissue, usually muscle or subcutaneous adipose tissue. The probe has a semipermeable membrane at its tip and is constantly perfused with a physiologic solution at a flow rate of of 1.5 to 2.0 µL/minute. Antimicrobials within the tissue diffuse out of the extravascular fluid into the probe, resulting in a concentration in the perfusion medium. However, the diffusion may be incomplete and depend on flow rates, temperature, membrane surface and porosity, and may not be constant over time (26). Therefore, calibration is required, usually using the retrodialysis method, which assumes that the process of diffusion is equal in both directions. The antimicrobial being studied is added to the perfusion medium and its disappearance rate through the membrane is calculated. In vivo percent recovery is then defined as
100 – [100 • (dialysate concentration out/dialysate concentration in)]
In vivo recovery differs markedly among differing antimicrobials (25). The absolute concentration in the interstitial space is calculated by the following equation:
100 • (concentration in the dialysate/percent in vivo recovery)
Since the semipermeable membrane excludes protein-bound drug, the technique measures free interstitial drug concentrations.
ANTIMICROBIAL ASSAY OF EXTRAVASCULAR FLUIDS AND TISSUE
The determination of antimicrobial agent concentrations contained in tissues can be accomplished by methods that are similar to those used for the determination of antibiotics in body fluids (see Chapter 8). The most readily available assay system at the present time is a microbiologic method. This system is based on an indicator organism that is very sensitive to the antibiotic under study and requires no special equipment. It allows for processing of large numbers of samples with minimal expense. An important aspect of this method is preparation of the standard concentration of antibiotic in the same tissue as the unknown sample (14,27). This is necessary so that drug binding to sample constituents is the same in both the unknown sample and the standard curve. Other methods also available for determination of antimicrobial concentrations include (high-pressure) liquid chromatography as well as many specific antibody-based systems such as radioimmunoassay (28) and enzyme immunoassay (29). These other methods, notably liquid chromatography and radioimmunoassay, require extraction of the antimicrobial agent from the specimen prior to assay. Use of these methods necessitates standardization with the tissue and antibiotic under study to ensure that all of the antimicrobial is extracted from the tissue sample or fluid prior to assay.
Microbiologic Assay
Antimicrobials can be determined using the bioassay method with only a limited number of organism species and a few selected media. The agar diffusion bioassay method described by Sabath (30) using Bacillus subtilis American Type Culture Collection strain 6633 is demonstrative of the technique for assay of antimicrobial compounds. The bioassay relies on an antibiotic-sensitive indicator organism seeded into an agar base on to which paper disks containing antibiotic are placed. Tissue homogenates or body fluids may also be placed on the agar in cylinders or in wells that have been cut into the agar. The cylinder and agar-well techniques usually provide increased sensitivity for the bioassay method. The antibiotic in the sample then diffuses into the agar and inhibits growth of the indicator organism. The diameter of the zone of inhibition is proportional to the concentration of antibiotic in the sample. Multiple variables can affect the diameter of the zone of inhibition. These variables include the indicator organism chosen, the agar base used, and the nature of the sample itself. Most of these variables can be eliminated by preparing the samples for the standard curve determination in the same type of tissue homogenate or body fluid as the specimen to be assayed.
Once the indicator organism and specific medium are selected, the microorganism is grown overnight and added to molten agar at 50°C. The microorganisms and agar are thoroughly mixed and this mixture is then poured into flat Petri dishes (15 × 100 mm) on a level surface. Each Petri dish contains a measured volume of 6 mL of the bacteria-seeded agar. The plates are left at room temperature on a level surface to harden and are then stored at 4°C until used. Plates should be stored for a maximum of 14 days under these conditions. The standard line is prepared by adding known concentrations of antibiotic to either tissue fluid or tissue homogenate and subsequently making serial dilutions. Samples of both known and unknown antibiotic-containing fluid are then placed on filter paper disks (usually 20 µL per disk) or pipetted (20 to 200 µL) into cylinders or wells cut in the agar surface. If wells or cylinders are used, the sample should be allowed to diffuse into the agar before movement of the plates to the incubator is attempted. The plates are incubated for 24 hours at 37°C and the diameter of the zone of inhibition is read with calipers to the nearest 0.1 mm. All samples should be assayed in triplicate to ensure an error rate of no more than 10%. The standard line is then plotted on semilogarithmic graph paper and the concentration of antimicrobial in the specimen is read from the standard line.
Occasionally, combinations of antimicrobials can be assayed using this method; however, it is not recommended because the influence of combined agents on indicator microorganisms is often unpredictable. Inactivation of aminoglycosides can be accomplished by lowering the agar pH to 5.5 or less. Also, penicillins or cephalosporins can be inactivated by the addition of β-lactamases to the specimen. When this is done, the same procedure should be performed on all standards to ensure the accuracy of the results.
Liquid Chromatography
Liquid chromatography for the measurement of antimicrobial agent concentrations is a specific method for assay of these drugs. The technique measures drugs in a liquid mobile phase by separating them from interferences on a column under high pressure and then using light absorption for agent detection. The technique is able to separate antimicrobials from other compounds in the sample as well as from both serum and tissue interferences. The method has been reviewed by Yoshikawa et al. (31). Extraction of antimicrobial compounds from the specimen is critical for this assay method. Because of the high protein content in most specimens, direct injection onto the liquid chromatography column is not recommended because the proteins plug the column, thus diminishing its efficiency and useful lifetime. Deproteination of samples can be accomplished by use of organic solvents or acids. Methanol, acetonitrile, and trichloroacetic acid are commonly used for this purpose.
After mixing the specimen with these agents, it is necessary to sediment the protein by centrifugation of the specimen and then inject the supernatant into the chromatographic equipment. Extraction has also been performed by adsorption of the specimen with silica gel and by ion-exchange chromatography for aminoglycosides. We have described a method using ion-exchange chromatography for extraction of penicillins and cephalosporins as well (32–34). Liquid chromatography has the advantage of specifically identifying the compounds under study by their pattern of retention on the chromatographic column. The disadvantages are that the extraction procedures and chromatography time required limit the number of specimens that can be assayed in one 24-hour period. The applicability of liquid chromatography for separation of antimicrobial drugs either singly or in combination is extensive. Once extraction techniques and detection methods have been standardized, this method can quantitate virtually any agent. Liquid chromatography is also very precise and highly reproducible, with coefficients of variation usually less than 5%. The sensitivity of liquid chromatography is similar to that of microbiologic assays and is satisfactory for both clinical and research use. Liquid chromatography with tandem mass spectrometry (LC-MS/MS) has the advantages of high sensitivity and specificity with short analysis time, which is advantageous in analysis of unstable drugs (35).
Antibody Methods
These methods of antimicrobial assay are based on the availability of specific antibody that binds the drug. The amount of drug bound is then measured either by radioactivity or by the availability for enzymatic reaction (28,29). Antimicrobial detection with radioimmunoassay is generally available for the aminoglycosides. The assays are rapid and specific and are as sensitive as microbiologic assays. The radioimmunoassay requires an extraction step; however, the enzyme immunoassay techniques are very rapid and have the advantage of not requiring an extraction. Their usefulness is limited by the availability of specific antisera and thus far, they have been applied to assays of aminoglycosides, vancomycin, and chloramphenicol. The antibody-based methods have been used little for antimicrobial assays of tissue homogenates and therefore require considerable standardization before being applied to assays of drugs in tissues.
PREPARATION AND INTERPRETATION OF SAMPLES COLLECTED FROM EXTRAVASCULAR FLUIDS AND TISSUES
The accurate determination and interpretation of antimicrobial assay results from extravascular sites are difficult and often confusing. The tables presented in this chapter illustrate the need for careful sample preparation and standardization in order to avoid conflicting results and possible misinterpretation of data.
Sampling of whole tissues for antibiotic assays in excised tissue should be performed in a carefully controlled manner. The area to be sampled should be identified at operation and easily visible. If possible, the arterial supply should be transiently clamped to allow blood to flow out of the prospective sample area. The specimen is then surgically removed and freed of adherent blood by gentle pressure with sterile gauze. Tissue samples should then be either immediately processed or stored at −70°C until antibiotic assay can be performed. Tissue fluid can be obtained from the tissue sample by homogenization in a phosphate-buffered saline solution at neutral pH. It is usually necessary to dilute the specimen with three volumes of buffer for every one volume of sampled tissue. The homogenization should be done in a hand-homogenizer so that temperature in the specimen is not elevated, which may destroy some drugs. The resulting homogenate can then be assayed directly for antibiotic content as well as for markers of various tissue compartments and blood. The homogenate can also be centrifuged and the supernatant assayed. We suggest preparation of antibiotic solutions for the standard line at the same time as preparation of the unknown sample so that the standards and the unknown are exposed to the tissue for the same amount of time under the same conditions (27).
Rauws and Van Klingeren (36) and Bergan (37) have discussed the difficulty in interpretation of antimicrobial tissue concentrations obtained from homogenized whole tissues. The final amount of detected drug is the sum of the concentrations of the individual components of the tissue as given by:
CT = Cp fb(1 − Ht) + Ce fb(Ht) + Ci fi + Cc fc + Cs fs
where C is the concentration in whole tissue (CT), plasma (Cp), erythrocytes (Ce), interstitial fluid (Ci), tissue cells (Cc), and specialized tissue components (Cs) such as secretory fluids, and f is the fraction of the homogenate contributed by blood (fb), interstitial fluid (fi), tissue cells (fc), and specialized tissue components (fs). Ht is the hematocrit level, a critical determination for most studies. The sum of the homogenate fractions is equal to 1, that is, fb + fi + fc + fs = 1. Because the contribution of antimicrobial agent in blood is one of the most important potential inaccuracies in the assay of tissue homogenates, correction for blood contamination is essential. We have indicated in the tables whenever this has been done. The formula of Plaue et al. (38) can be used for this correction. Their formula simply states that extravascular concentration = (tissue concentration − serum concentration)/(tissue water volume − blood volume).
Blood contamination can be estimated most simply by measurement of the hemoglobin content of the tissue homogenate and comparison of this with the hemoglobin content of the subject’s circulating blood. If estimation of blood contamination is not possible, the significance of this factor can be minimized by obtaining the extravascular fluid or tissue sample at a time when the intravascular antibiotic concentration is expected to be low or at least lower than that in the extravascular site under study. Rinsing the specimen to remove blood should also be avoided because most antimicrobials at the low concentrations found in vivo are readily water-soluble and easily washed out. If desired, the extracellular or interstitial fluid in tissue can also be measured with high-molecular-weight dextran, sodium bromide, or sodium thiocyanate, which is excluded from intact cells (e.g., kidney, gallbladder, and prostate). Corrections for antibiotic concentrations of the secretory product also need to be considered because these fluids often contain high concentrations of antimicrobial agent and significantly contaminate tissue specimens. Most published work on tissue distribution of antibiotics fails to make even simple adjustments for blood contamination, making interpretation difficult or impossible.
Finally, because various body tissues bind antimicrobial agents with different capacities (39) and tissue fluid–antibiotic concentration is a combination of bound and unbound drug (40), it is necessary to know the protein binding of an antibiotic to both the tissue or body fluid and plasma or serum in order to interpret correctly the significance of the tissue concentration. Methods for measurement of protein binding to serum, tissues, and body fluids have been published (39,41,42) and measurements should be performed to optimally interpret extravascular fluid and tissue antimicrobial concentrations.
INTERPRETATION OF EXTRAVASCULAR ANTIMICROBIAL DISTRIBUTION
The proper interpretation of an extravascular antimicrobial concentration depends on a series of factors that are often overlooked or ignored (Table 14.2). These factors (with the possible exception of protein concentration) are independent of permeability barriers or effects of inflammation that may influence the amount of antimicrobial that reaches a given site. They also do not take into account the antimicrobial susceptibility of pathogens likely to be found within a given extravascular site. Such comparisons require a higher level of cognition and are left to readers who may wish to correlate minimal inhibitory concentrations for specific organisms found elsewhere in this text with extravascular site drug concentrations found at the end of this chapter (43). Readers who make such an effort are cautioned that expectations of clinical cure or failure should not be made with a high degree of confidence until more clinical studies on this subject are available (44,45).
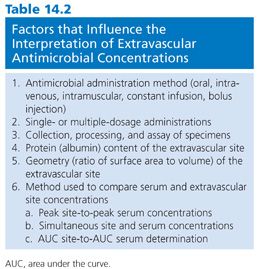
Administration Method
The method used to administer an antimicrobial can result in marked differences in serum concentration. High concentrations in serum result rapidly from bolus injections and influence the rate at which antimicrobials enter an extravascular site (46,47). Similarly, intermittent administration results in more rapid attainment of high extravascular levels than does constant intravenous infusion (48,49). Furthermore, extravascular drug concentrations are frequently compared with peak serum concentrations. Rapid intravenous infusions can result in very high serum peaks that, when compared with extravascular concentrations, may give the erroneous perception of poor extravascular drug penetration.
Single or Multiple Dosages
The concentration of antimicrobial at an extravascular site is influenced by the number of doses administered, particularly if the administration frequency is short relative to the serum half-life of the drug (50). Multiple doses may result in considerably higher extravascular antibiotic concentrations than do single doses (51), as a drug accumulates over multiple half-lives depending on the dosing interval. This gradient may be reduced if a loading dose is administered, but the level achieved by a loading dose may not equal the result of the multiple half-lives of accumulation required to reach steady-state condition.
Specimen Handling
Different methods of processing and assaying specimens may lead to confusion in interpretation of extravascular levels, particularly in whole tissues. As previously discussed, the method used to remove blood from tissues, the assay method and standards, and blood contamination corrections are all important to the interpretation of extravascular antibiotic concentrations. Comparison of studies that use dissimilar handling methods is extremely difficult.
Extravascular Protein Content
Antibiotics often bind to proteins (usually albumin) at the extravascular site as well as in serum. For highly bound antimicrobials, the amount of drug that accumulates in an extravascular space depends on the protein content of the space. A site with high albumin content binds a substantial amount of a highly bound antibiotic, and the total (free plus protein-bound) antibiotic concentration in the site is greater at equilibrium than it would be for a site with low albumin content (7,40). In contrast, a site that contains little albumin may have a low total antibiotic concentration, but most of the antibiotic present is free (unbound) drug.
Site Geometry
The amount of antibiotic in a fluid-filled space largely depends on diffusion and, in turn, on the surface area available for diffusion compared with the volume of the space, the so-called surface area to volume (SA/V) ratio. It has been shown in experimental models that spaces with high SA/V ratios show fluctuations of antibiotic concentrations that parallel serum fluctuations, while spaces with low SA/V ratios show a damped response with lower peaks and higher troughs than in serum (52). Animal models that use tissue cages and chambers as extravascular sites and human blister models of extravascular distribution are subject to variation in drug penetration because of differences in SA/V ratios (52). This simple ratio of SA/V is the major determinant of peak and trough drug levels in large-volume, fluid-filled spaces that fill mainly by passive diffusion. Experimental data for ascites in dogs clearly support this concept (53).
Method of Serum and Site Comparison
The method used to compare serum and extravascular site antibiotic concentrations can markedly influence the perception of how well a drug enters an extravascular site. Most antimicrobials are administered intermittently, yielding a rapid rise in serum concentration followed by a logarithmic decay in serum levels. Extravascular site concentrations follow a similar pattern but peak levels are usually lower and occur later than in serum, while trough levels are higher than those in serum (Fig. 14.1). Comparisons of serum and site concentrations are usually done in one of three ways: peak-to-peak comparison, simultaneous-time comparison, or AUC-to-AUC comparison. Peak-to-peak comparisons are difficult to obtain clinically because large numbers of specimens are required to ensure that the peak is obtained. In addition, serum peaks may be very high following rapid intravenous infusion, resulting in an inappropriately low ratio of site-to-serum concentrations. Simultaneous-time determinations are most often employed clinically because it is simpler to obtain a serum specimen at the same time that the site is sampled. Because serum levels are falling at the same time that site levels are rising, the perceived penetration of an antibiotic into an extravascular site may be low if specimens are taken shortly after administration (high serum level and low site level) but appear much higher if sampling is delayed until serum levels have fallen and site levels have risen. Perhaps the best way to compare serum and site antibiotic penetration is by means of AUC data. These studies also require multiple serum and site samples and thus are difficult to perform in certain sites in humans. They are best done following multiple antimicrobial doses to allow equilibrium to occur.
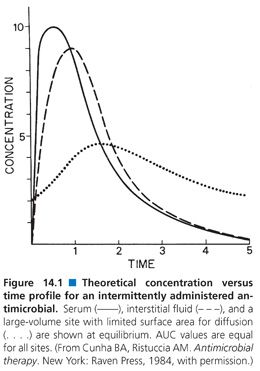
TABLES AND COMMENTS
General Comments
Tables 14.3 to 14.20 have been prepared from published literature for reference purposes to illustrate the concentrations of antimicrobials found in a variety of human extravascular sites. Sites have been selected to be representative of each of the categories listed in Table 14.1. Thus, the sites include fluid-filled spaces such as ascites, pleural effusions, joint fluids, and cutaneous blisters (as well as cutaneous chambers, disks, and threads); secretory fluids such as bile, sputum, breast milk, middle ear fluid, and prostate and sinus secretions; barrier spaces such as aqueous humor and CSF; and the whole-body tissues bone, skeletal muscle, cardiac tissue, gallbladder, lung, and prostate.
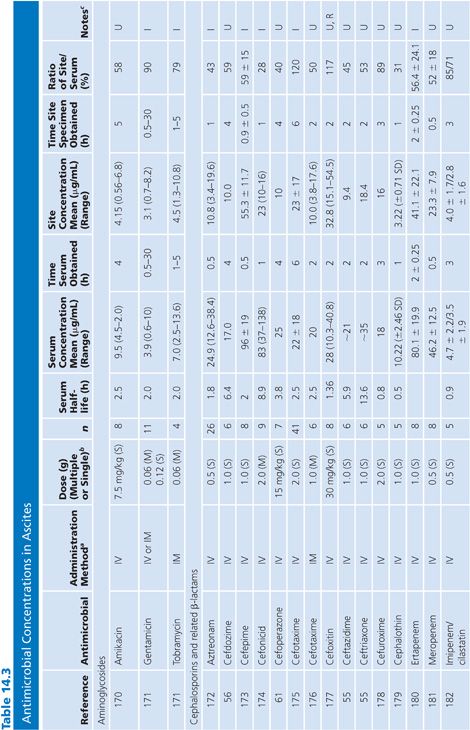
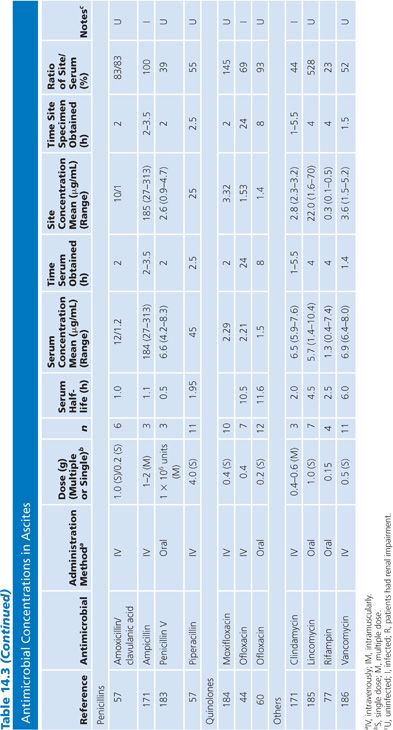
Requirements for inclusion of a reference in the tables were that the article supply information about drug dosage; number of doses; concentrations of drug both at the site and in serum; timing of both the site and serum specimens; and, if possible, the range or standard error or standard deviation of the data. Only articles with data from at least three subjects were included. Data from articles were frequently reorganized to include only information for specific sampling times in order to make interpretation easier. Extrapolation of graphical data was occasionally required. Many articles contained far more data than could be included in these concise tables. In such cases, data from specific sampling times (e.g., 1, 2, or 3 hours) were arbitrarily selected to obtain simultaneous-time data for the tables. Certain antimicrobials have been studied numerous times at certain body sites. In these instances, no attempt was made to include every reference in the tables. Rather, emphasis was placed on inclusion of data about as many different drugs as possible.
Antimicrobials are grouped into aminoglycosides, cephalosporins and related β-lactams, penicillins, quinolones, tetracyclines, and other antimicrobials. Included under cephalosporins are cephamycins and related β-lactams such as moxalactam, monobactams, and carbapenems. All antimicrobials are listed alphabetically within categories. Method of administration, dose (in grams), and whether single or multiple doses were given are listed after the drug name. The number of subjects studied to obtain the data in the table is given in the column headed n. Serum half-life, if not supplied in the references, was obtained from standard tables (54). The remainder of the table gives the serum concentration (micrograms per milliliter or units per milliliter for penicillin) and time obtained, followed by the site concentration (micrograms per milliliter or micrograms per gram of tissue) and time obtained. The ratio of site concentration to serum concentration is given as a percentage. The “Notes” column includes special information about whether the site was infected or uninfected (if the information is known), whether site assays were corrected for blood contamination (a rarity), and any special information needed to interpret a given study. Specific comments regarding each table are included in the following sections.
Ascites
There are only limited data on antibiotic penetration into ascitic fluid. Data in Table 14.3 are limited to patients from whom ascitic fluid could be aspirated. Only studies that included fluid obtained by aspiration are included. Studies that obtained fluid by placing a paper disk under a loop of bowel at surgery or that measured peritoneal fluid samples from peritoneal drains after abdominal surgery or from patients undergoing peritoneal dialysis were not included. In the majority of studies, antibiotics penetrated into ascitic fluid well, although there was a lag in the ascitic fluid values compared with the serum values in the first few hours after the initial dose. AUC ratios of ascites to serum for several antimicrobial agents of different classes ranged from 30% to greater than 100% (55–61).
Pleural Fluid
The antibiotic levels in pleural fluid are listed as infected (i.e., empyema) or uninfected (Table 14.4). The uninfected groups consist of patients with congestive heart failure and malignant or parapneumonic effusions; some also included cases of nonpyogenic infection such as tuberculosis. When specified, most samples were collected by chest tube drainage. Few studies provided data on antibiotic penetration into pleural empyemas. It is notable that the aminoglycosides penetrated poorly into empyema fluid, compared with uninfected pleural fluid, as shown by the study of Thys et al. (62). The quinolones demonstrated good pleural fluid penetration, which appeared to be enhanced by the presence of empyema. Aztreonam also demonstrated excellent penetration into the pleural fluid.
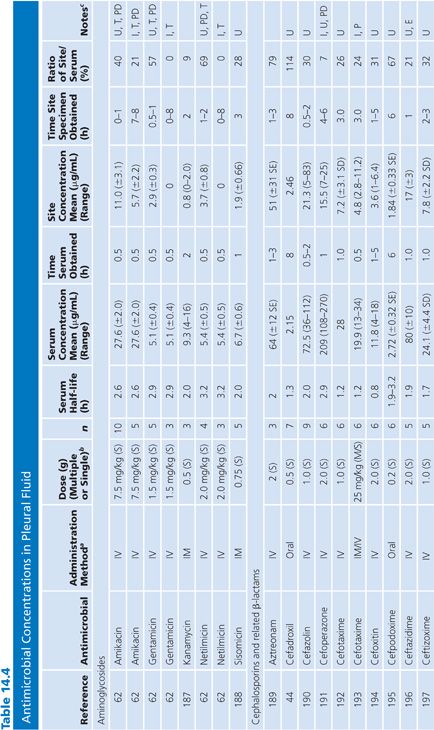
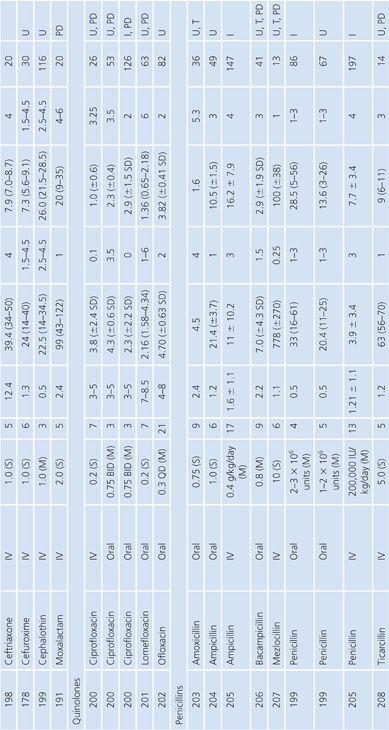
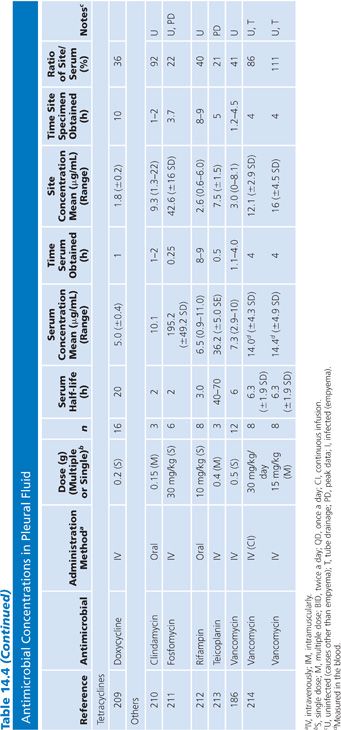
Joint Fluid
In compiling this table of antimicrobial penetration into joint fluid spaces (Table 14.5), single-patient studies were combined when possible to give a better overall reflection of the penetration of individual agents. No attempt at correction for blood contamination was made, and only rarely was there an attempt made to correlate protein content or antimicrobial/tissue fluid protein binding with drug penetration (24). The reported fluid-to-serum antimicrobial concentration ratios are quite high for this group of studies, which is primarily due to the high protein content of the extravascular fluid plus the fact that many of these studies were performed after multiple systemic doses of the antimicrobial agent had been administered. Data from single infected patients treated with cephapirin (63) have not been included in Table 14.5.
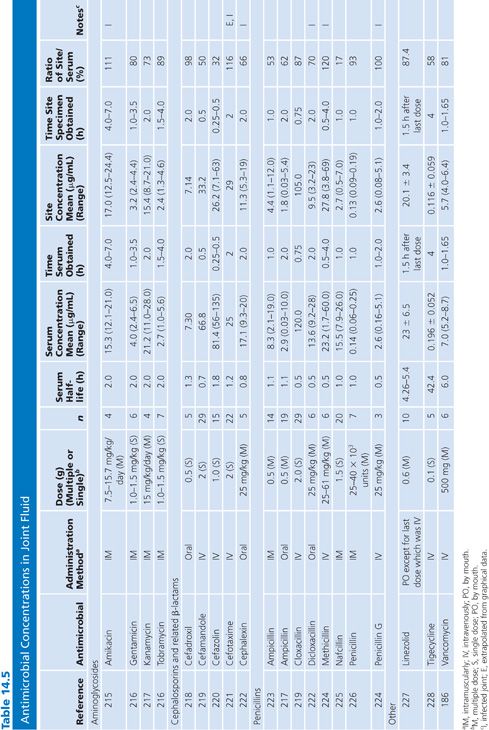
Cutaneous Blister/Disks/Threads/Microdialysis Samples of Interstitial Fluid
Table 14.6 contains a summary of reports of antimicrobial distribution based on models in humans. All sites were done in uninfected patients except the studies done by Tegeder et al. (64) of imipenem. None of the studies have made any attempt to correct for blood contamination other than avoiding gross blood. Data are also available for penicillin (23) and pivampicillin (23) but are not included in Table 14.6 because fewer than three subjects were studied. While only a limited number of multiple-dose studies have been done, even the single-dose studies often demonstrate high ratios of drug concentration in the extravascular site to that in serum, reflecting the small size of the extravascular space under study. One study attempted to correlate protein concentration to drug penetration and found a significant correlation at r = 0.97 (22). There was considerable variability in the ratio of extravascular to intravascular antimicrobial concentrations, reflected by ratios as low as 3% for nafcillin in the skin-window model (15) to 238% for FCE22101 in a cantharides blister study (65). Many articles supply data that can provide an AUC for the agent under study in both the intravascular and extravascular spaces. This is a notable achievement in the study of extravascular drug penetration, although more multiple-dose studies are needed as well as more attention to antimicrobial binding at extravascular sites.
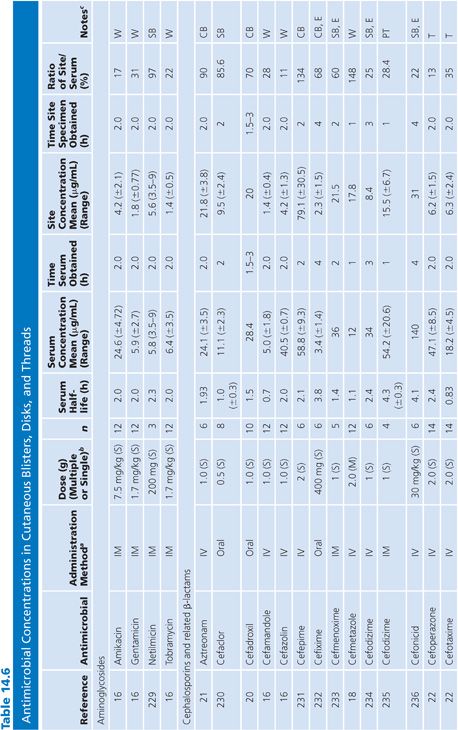
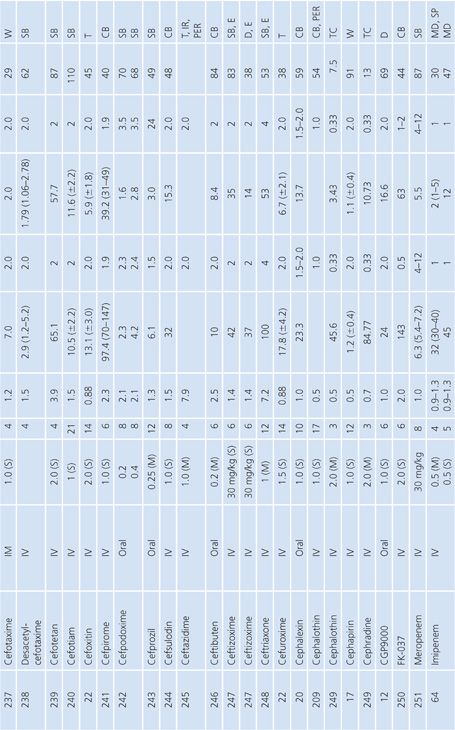
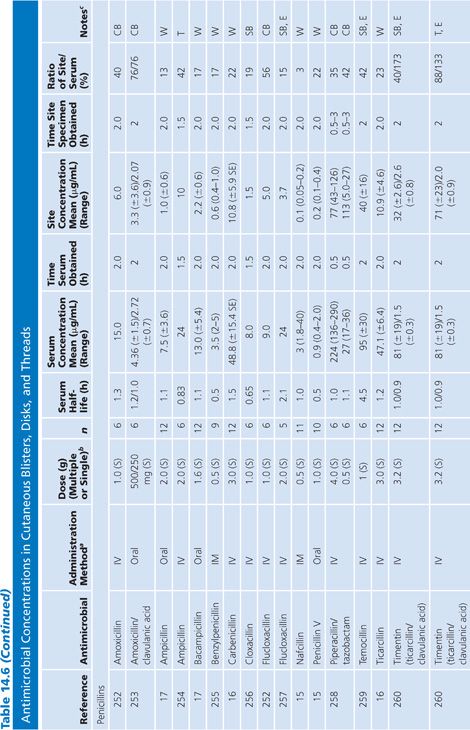
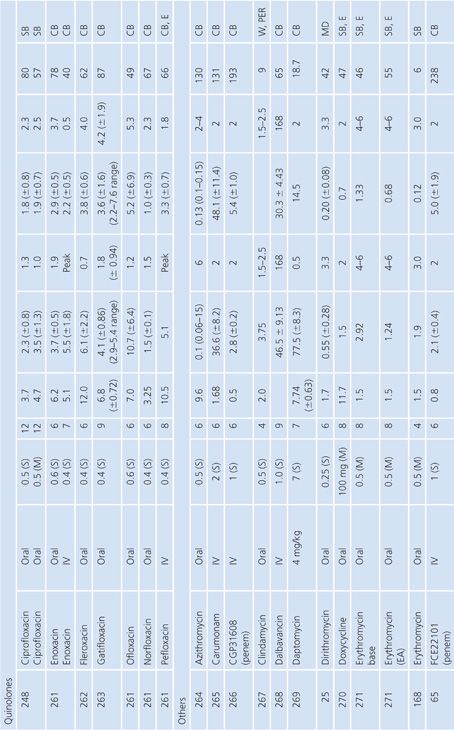
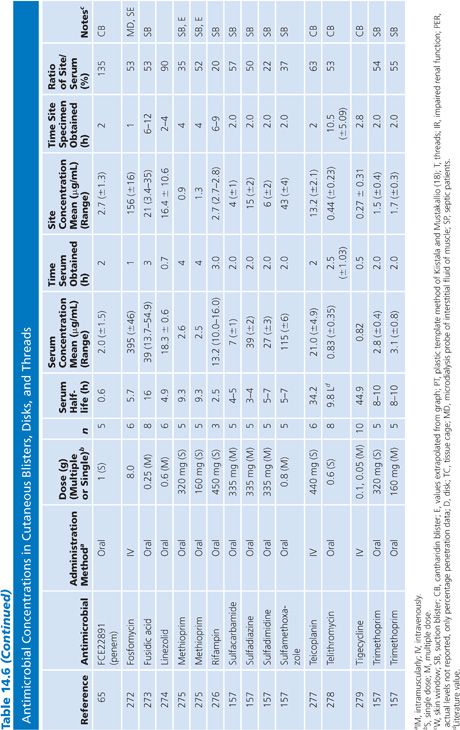
Bile
Specimens of bile obtained by three methods are included in Table 14.7: aspirations of gallbladder or common duct bile at surgery, external bile drainage from T-tubes, or bile obtained by endoscopic retrograde cholangiopancreatography. Studies in which bile was obtained by duodenal aspiration were not included because of unknown specimen dilution by gastrointestinal fluids. Several studies in Table 14.7 confirm the observation that bile concentrations are lower or absent in the presence of cystic duct obstruction (66) or common bile duct obstruction (67–69). In general, T-tube and common duct bile levels seem to be somewhat higher than gallbladder bile levels (70–74) when comparably timed specimens are obtained. This may be related to partial obstruction of the cystic duct. Multiple antibiotic doses also result in higher bile levels than do single doses (74–76).
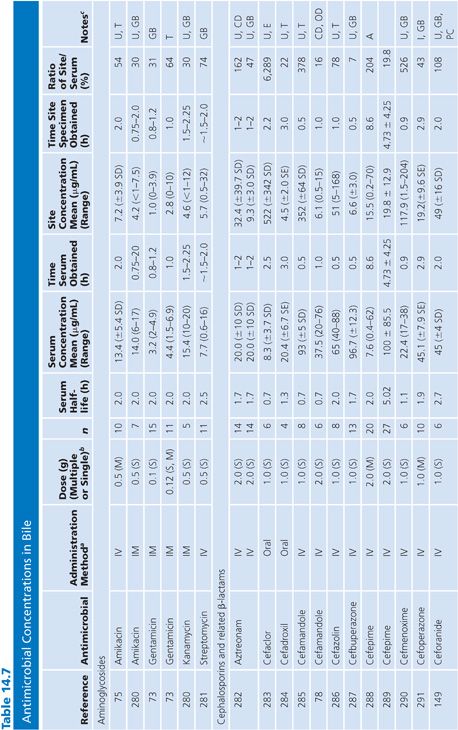
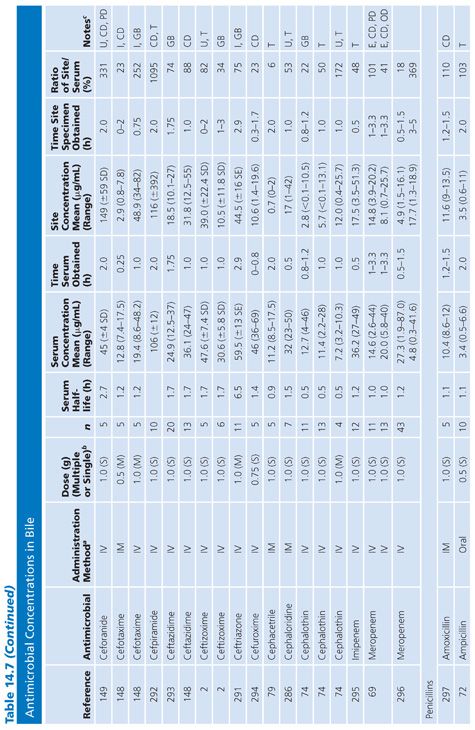
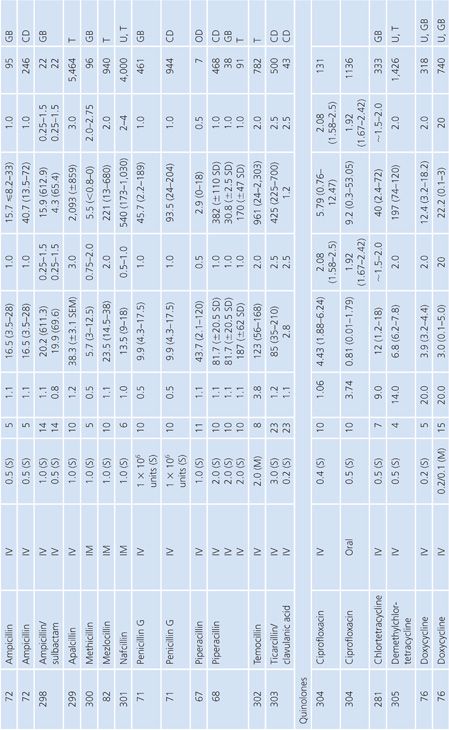
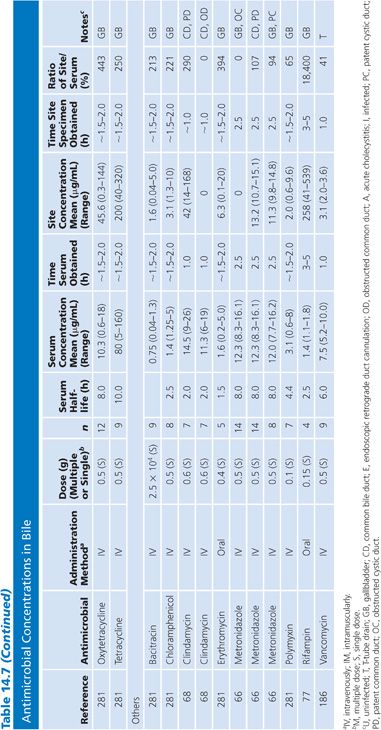
Ratios of site-to-serum levels are extremely variable, ranging from zero in the presence of obstruction to 18,400% for rifampin (77), with numerous examples of ratios greater than 100%. After relief of biliary obstruction, the excretion of antibiotics (aztreonam, piperacillin, and cefamandole) remains depressed for a prolonged period of time, which may exceed 28 days (67,78). Data on ratios of AUC in bile to AUC in serum were available for a limited number of drugs, and they also showed wide variation: 19% for cephacetrile (79); 38% to 44% for amoxicillin, penicillin G, and oral ampicillin (71,72); 46% for ceftazidime (80); 65% for amikacin (75); 96% for intravenous ampicillin (72); 100% for cefuroxime (81); and 2,089% for mezlocillin (82).
Although biliary penetration is often touted as advantageous in the therapy of biliary tract infection, it is not at all clear that this is an important treatment variable in view of the poor penetration in the presence of obstruction. Keighley et al. (83) have shown that biliary antibiotic concentrations have no influence on rates of bacteremia and wound infection associated with biliary tract surgery. Smith and LeFrock (84), Nagar and Berger (85), and Dooley et al. (86) have extensively reviewed biliary antibiotic penetration and clinical use in biliary infection.
Sputum
Both sputum and bronchial secretion specimens are included in Table 14.8. Bronchial secretions were obtained via bronchoscopy or by suction aspiration from patients with endotracheal tubes or tracheostomies. Some articles provide data on antimicrobial concentrations in epithelial lining fluid in the lung, which is indicated in Table 14.8 (87). Most subjects had chronic bronchitis, but some studies were done with children with cystic fibrosis, and occasionally patients had pneumonia. Nearly all studies were done with infected patients. Some studies suggest higher sputum antibiotic levels with the use of mucolytic agents (88–90). Ratios of sputum-to-serum concentrations are low for most drugs. Data on AUC ratios were limited but showed ratios of 2.7% and 4.5% for cefotaxime (91), 14% for cefuroxime (92), 106% for ciprofloxacin (93), and 19% for netilmicin by both intermittent and continuous intravenous infusions. Sputum concentrations of the cefotaxime metabolite desacetyl-cefotaxime are much higher than those of the parent drug (94).
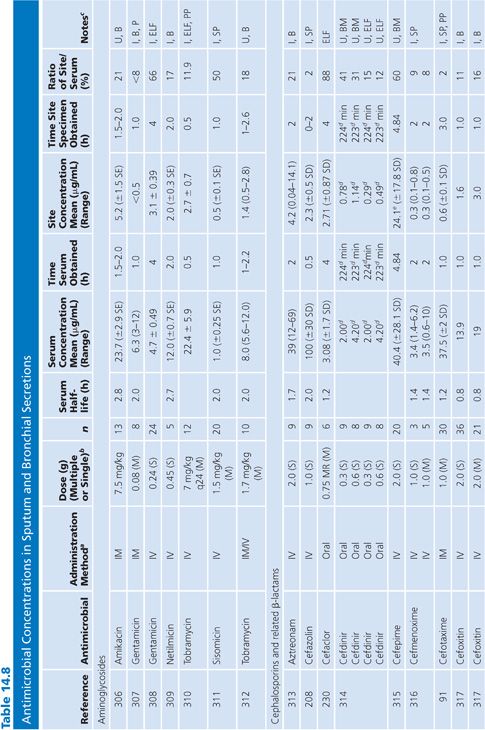
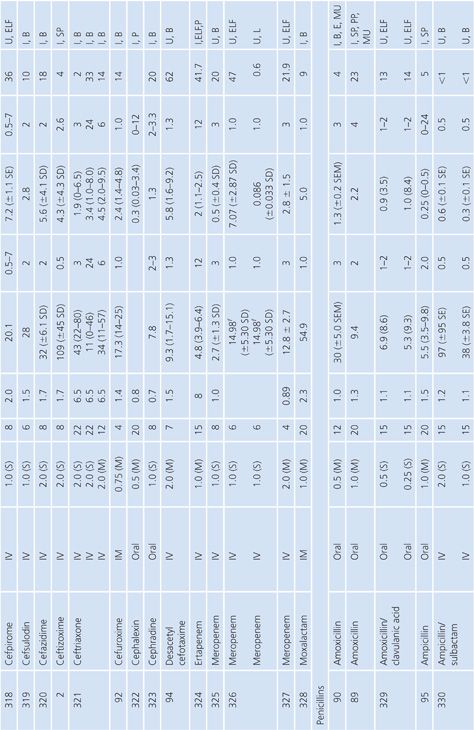
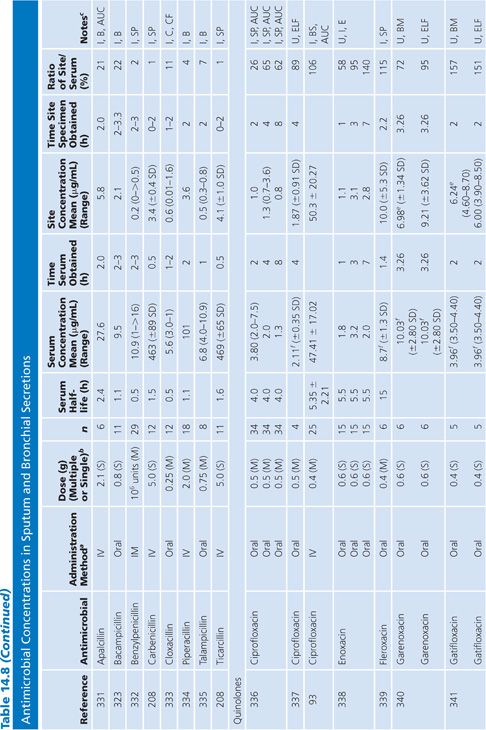
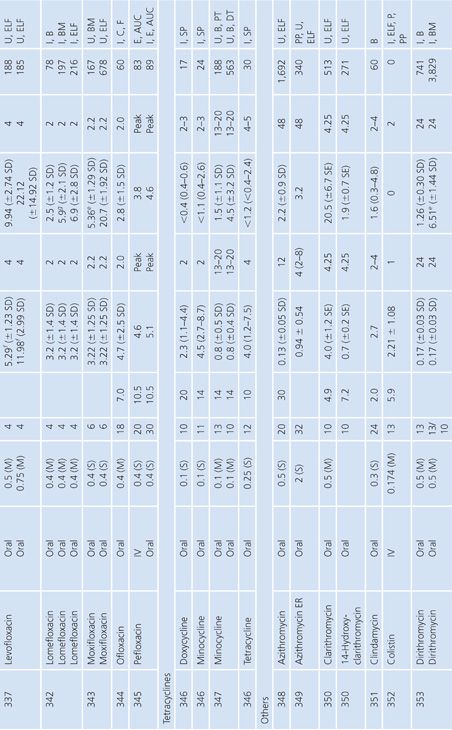
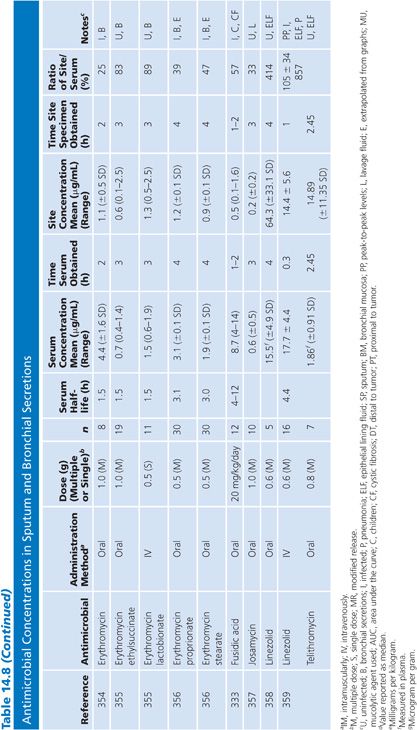
Some studies have shown a relationship between clinical infection cure and antibiotic concentration in sputum (89,95) and have shown that the antibiotic concentration increases with the degree of sputum purulence for at least some antibiotics (96). Reviews of this topic have differed on the importance of sputum antibiotic concentrations to the cure of infection, with Lambert (97) taking a somewhat skeptical view, while Pennington (98) has taken a supportive stand with which Smith and LeFrock (99) have concurred. Readers are also directed to an excellent study by Wong et al. (100), which could not be included in Table 14.8 because of the graphical presentation of the data. Hitt and Gerding (43) have compiled ratios of sputum antimicrobial levels to the minimal inhibitory concentration for 90% of common bacterial pathogens that cause bronchitis and pneumonia. These ratios may prove predictive of clinical outcome, but good clinical correlation is lacking.
Breast Milk
The studies of breast milk (Table 14.9) were performed in lactating mothers who were normal volunteers or were being treated for nonbreast infections. The results show that the secretion of most antibiotics into breast milk is insignificant. However, a few agents, notably metronidazole, chloramphenicol, tetracycline, and lincomycin, were found in milk at levels approaching the serum levels. Giamarellou et al. (101) reported a study showing that quinolones (ciprofloxacin, ofloxacin, and pefloxacin) also have excellent penetration into breast milk, posing a possible risk to newborn infants. In a single study addressing milk penetration during puerperal mastitis, concentrations of phenoxymethyl-penicillin were higher in the milk from the mastitic breasts than in the nonmastitic breasts, but serum concentrations were not determined for the patients with mastitis (102). Even though only small quantities of most antibiotics reach the breast milk, the potential still exists for allergy or toxicity in the infant.
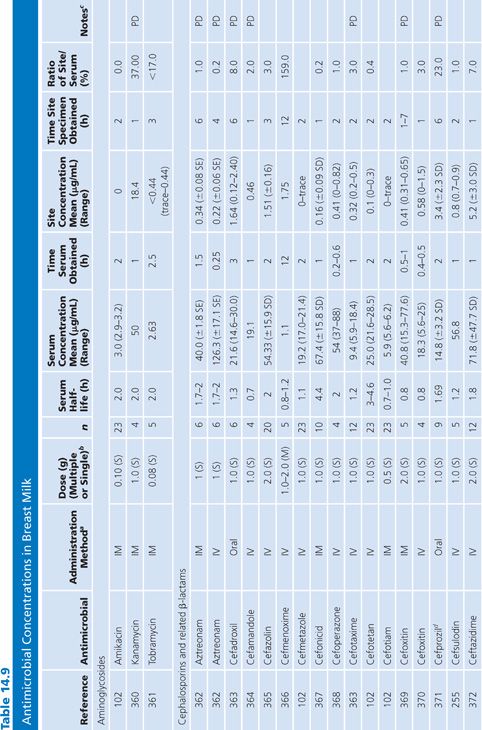
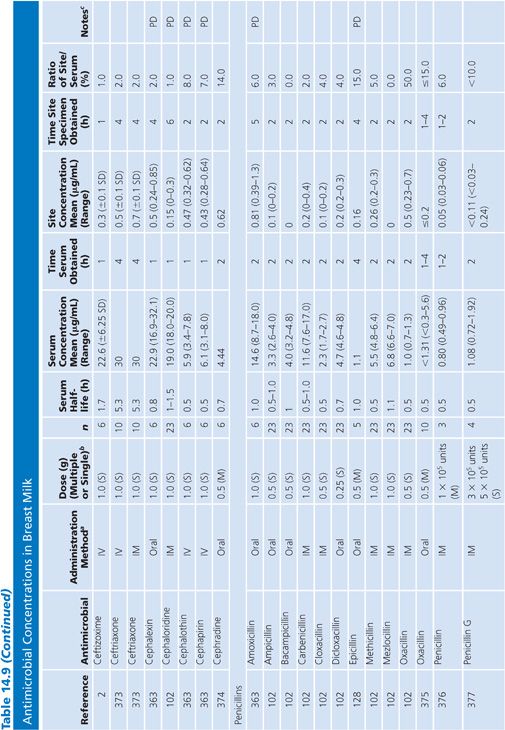
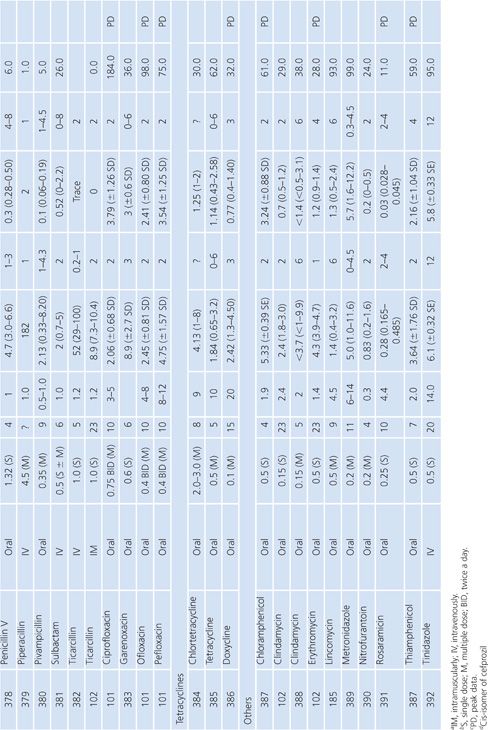
Middle Ear Fluid
Antibiotic levels were assayed in middle ear fluid from patients categorized as infected (acute otitis media) or uninfected (serous otitis or otitis media with effusion) (Table 14.10). The middle ear fluid samples were usually removed by needle aspiration. Pediatric subjects were studied most often. Most antibiotics penetrated significantly into the middle ear fluid. In the few cases where direct comparison was possible, the presence of acute infection appeared to enhance antibiotic penetration into the middle ear fluid.
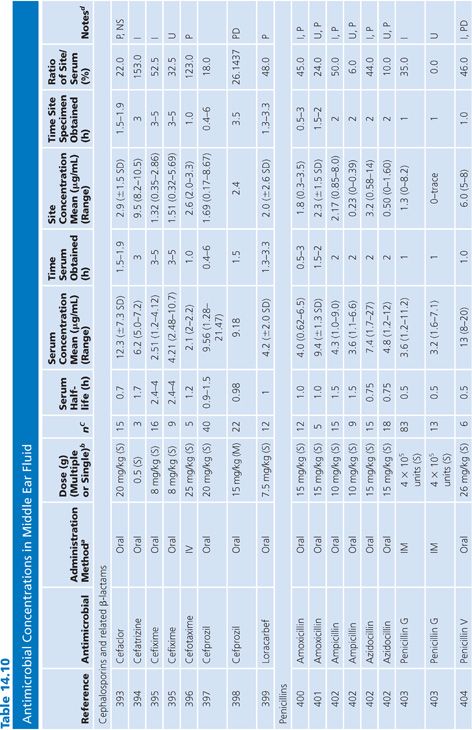
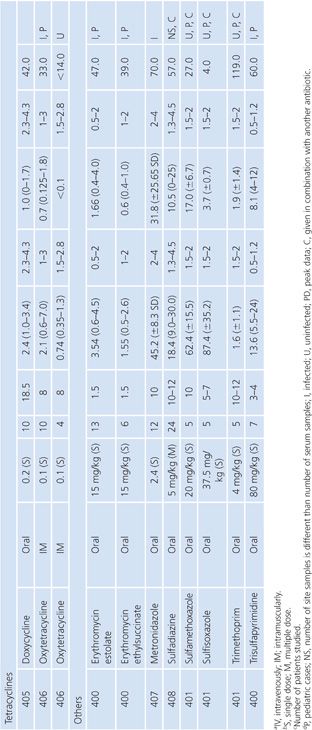
Sinus Secretions
The sinus secretion studies were done primarily in patients with either acute or chronic maxillary sinusitis. None of the studies corrected for blood contamination, but this was likely minimal. On the basis of the data presented in Table 14.11, tetracyclines, erythromycin, and trimethoprim seemed to achieve better penetration than the β-lactams. There may be an inverse correlation between degree of purulence and amount of antibiotic penetration (103,104). One clinical correlation showed that an antibiotic concentration higher than the minimal inhibitory concentration of the pathogen was associated with clearance of the bacteria (105).
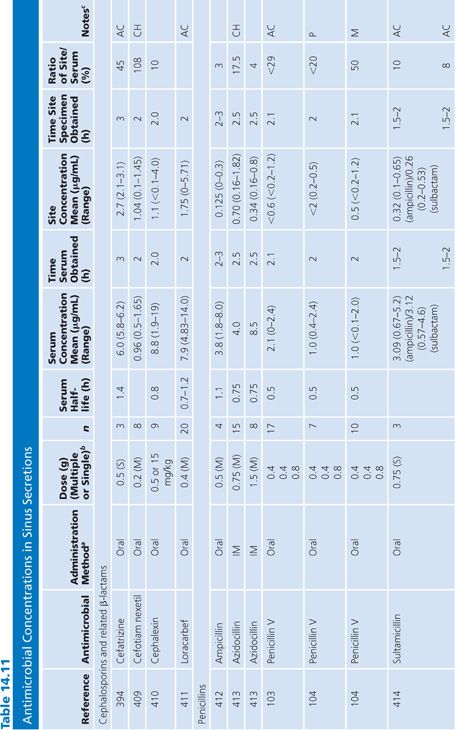
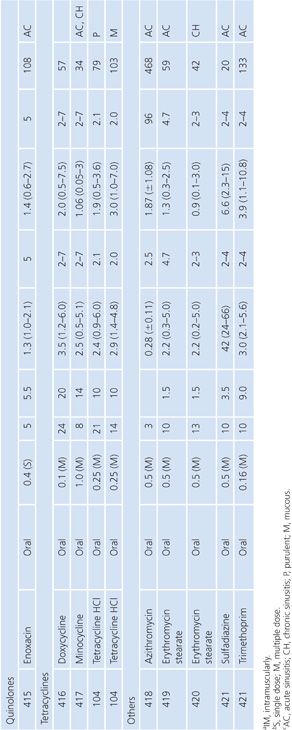
Prostatic Secretions
There are only a limited number of human studies detailing antibiotic concentrations in human prostatic secretions (Table 14.12). Winningham et al. (106) have determined in a dog model, in which urinary contamination has been excluded, that the major factors controlling antibiotic penetration into the prostatic fluid are the degree of lipid solubility, the pKa, and the amount of protein binding. Using the dog model, Madsen et al. (107), Meares (108,109), and Gasser et al. (110) have determined that only trimethoprim, erythromycin, rosamicin, oleandomycin, clindamycin, enoxacin, and fleroxacin concentrate into prostatic fluid. The dog model may not necessarily reflect the situation in humans, however, because prostatic secretions in humans with prostatitis are more alkaline than the secretion found in normal dogs (59,111). One study suggested that prostatic fluid concentrations of cephalosporins are increased in patients with acute prostatitis, compared with uninfected controls (112). The higher pH found in humans may favor the penetration of some quinolones that are zwitterions (110). The degree of antibiotic penetration into prostatic fluid may be more important than prostatic tissue concentration because bacteria that cause chronic bacterial prostatitis reside within the prostatic fluid, and the prostatic epithelium acts as a barrier to the passage of most antibiotics into the fluid (113). When prostatic fluid drug levels in normal patients were compared with levels in a patient with ureterosigmoidostomy, it was found that the drug concentrations of nitrofurantoin and ampicillin found in normal patients were almost entirely due to urinary contamination (114,115). One group measured iohexol in the prostatic fluid after intravenous injection to exclude urinary contamination (116–118). Clinical data have revealed that trimethoprim is only partially effective in the treatment of chronic bacterial prostatitis. Quinolones may be of some benefit in the treatment of chronic bacterial prostatitis, but long-term follow-up has often been inadequate (119).
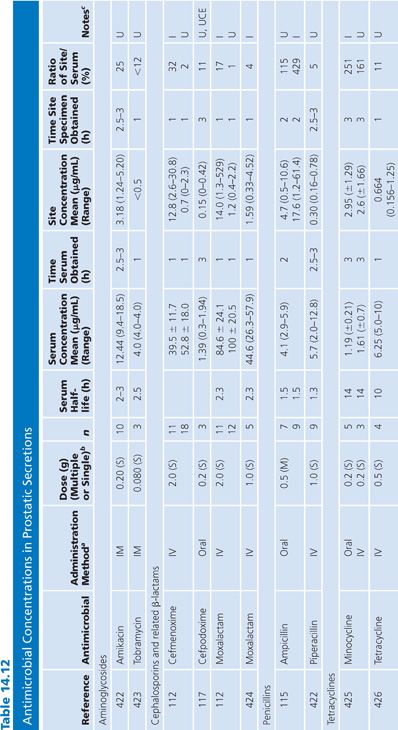
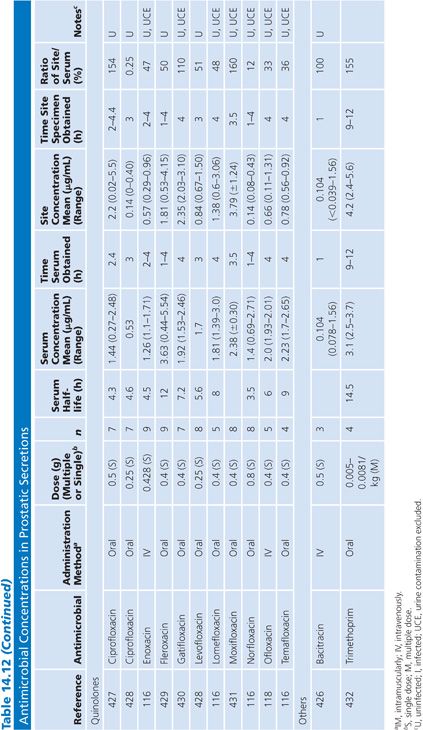
Cerebrospinal Fluid
Table 14.13 lists antimicrobial studies investigating the penetration of various agents into human CSF. There have been several reviews on the same topic that also dealt with the kinetics of central nervous system handling of drugs (120,121). There was no correction for blood contamination in these studies, and the results demonstrated considerable variability in the ratios of antimicrobial concentrations in CSF and serum. Many studies, typified by that of Mullaney and John (122), provide useful information on CSF levels of drugs such as cefotaxime, but make evaluation of drug penetration impossible by not presenting data on concentrations in serum. Similarly, data for ampicillin (123), nafcillin (124), and rifampin (125) are available but include small numbers of patients or lack actual drug levels. The CSF-to-serum ratio ranged from 0% for rifampin in uninfected controls (126) and 0% for low-dose oxytetracycline (127) to 199% for amoxicillin when measured 4 hours after a single intravenous dose (128). In general, because samples were taken at longer time intervals following the systemic drug administration, serum levels were lower in relation to CSF concentrations and the CSF-to-serum ratios increased. Data for several antimicrobials are included in detail to illustrate changes in CSF concentrations with time after administration, duration of meningitis, and multiple dosing (128–130).
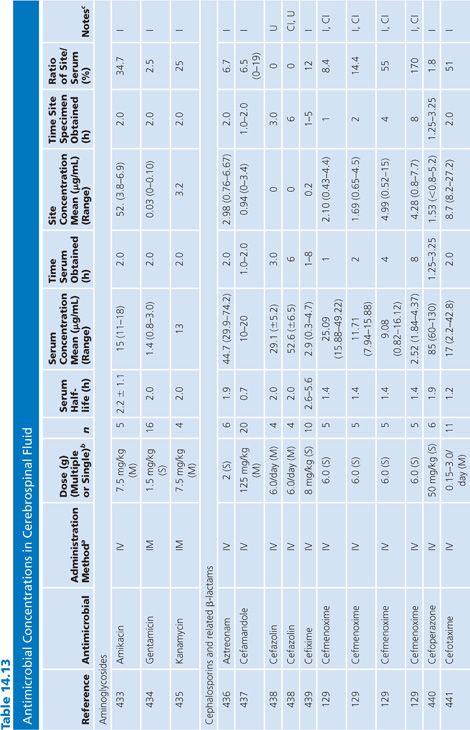
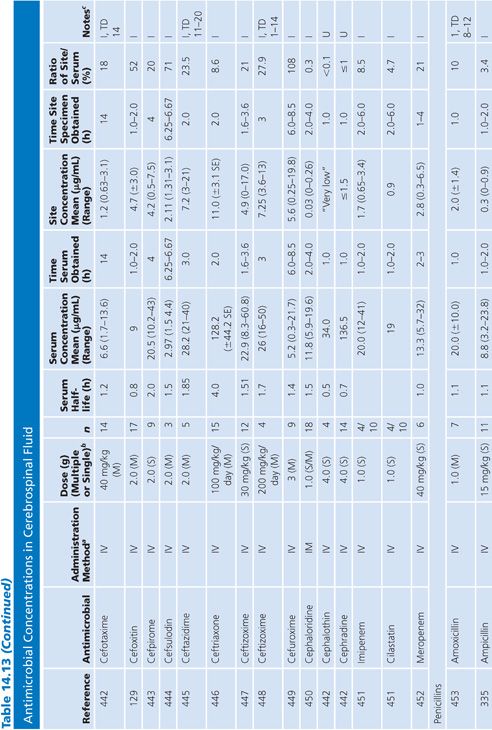
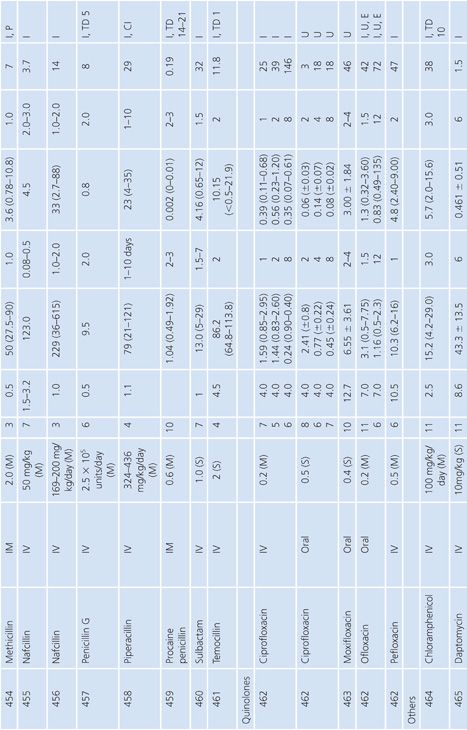
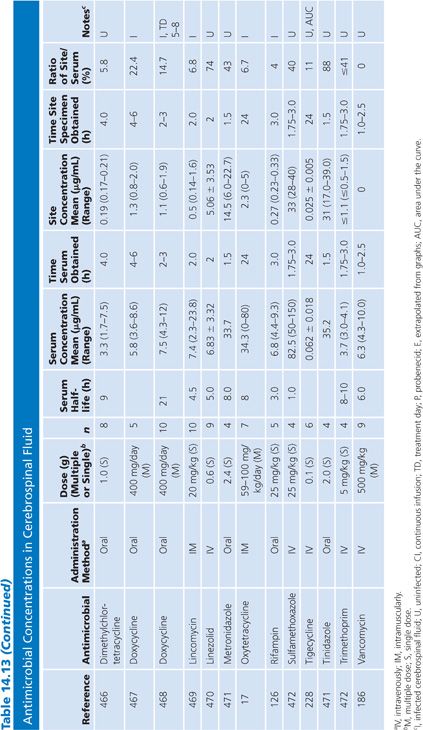
Aqueous Humor
In almost all studies, the antibiotic levels in aqueous humor were measured in uninfected patients undergoing cataract surgery (Table 14.14). Under those conditions, most antibiotics penetrated poorly into the aqueous humor when given systemically, resulting in levels that are inadequate for treating endophthalmitis. Data are limited on penetration of antibiotics into infected eyes. One study examined doxycycline levels in acutely inflamed eyes and found levels similar to those in uninflamed eyes (131). Animal studies of bacterial endopthalmitis have shown enhanced penetration into the aqueous and vitreous humors of infected eyes with some antibiotics (132,133).
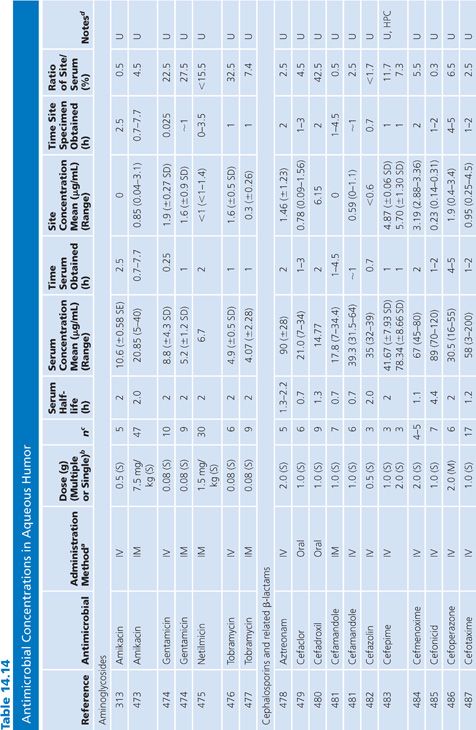
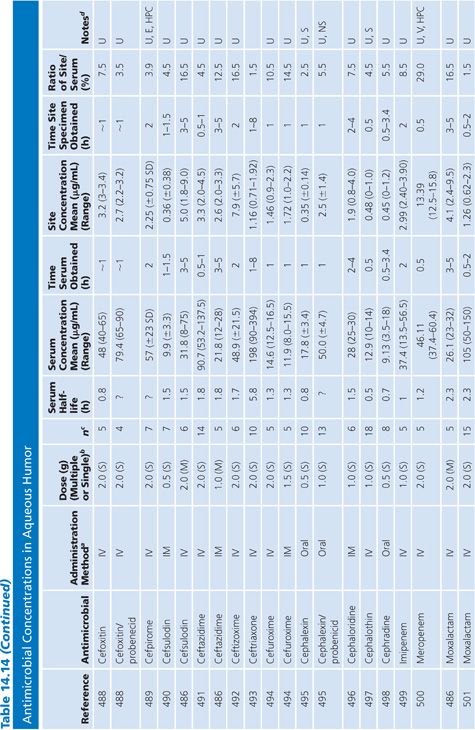
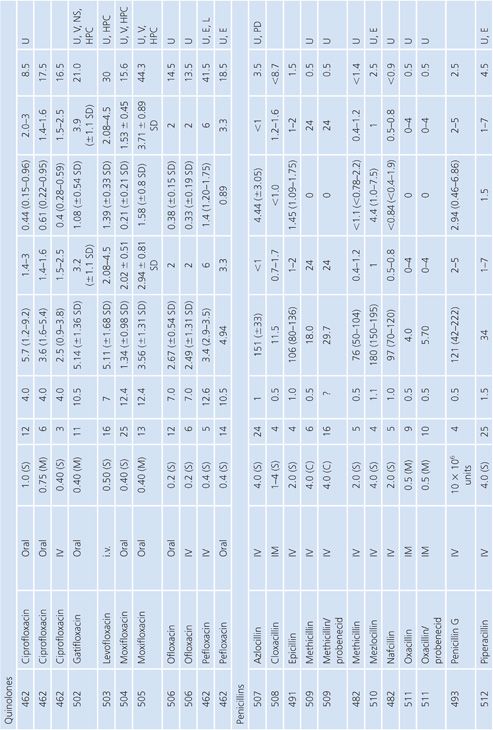
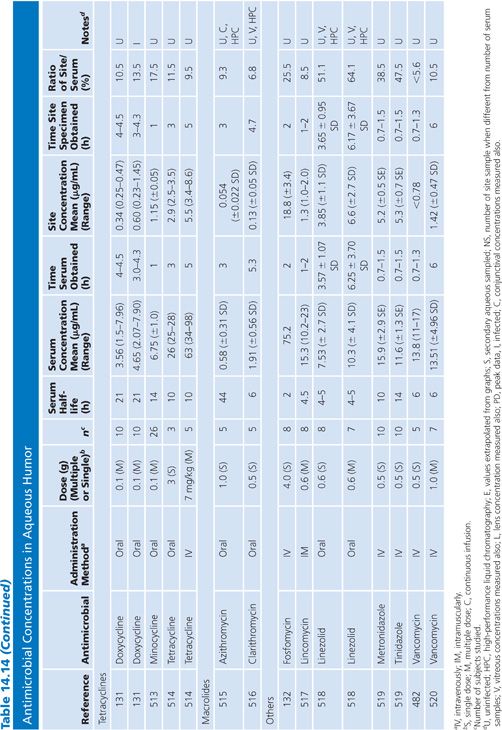
Skeletal Muscle
The majority of studies measuring antibiotic concentrations in skeletal muscle were done on uninfected normal muscle obtained during surgical procedures (Table 14.15). One study measured drug concentrations in the muscle of a decubitus ulcer (134). The higher concentrations observed for clindamycin and ciprofloxacin (compared with β-lactams) probably reflect the higher intracellular concentrations of lincosamides and quinolones (135). Newer studies have used microdialysis techniques, which distinguishes whole tissue from interstitial fluid concentrations within muscle (136,137).
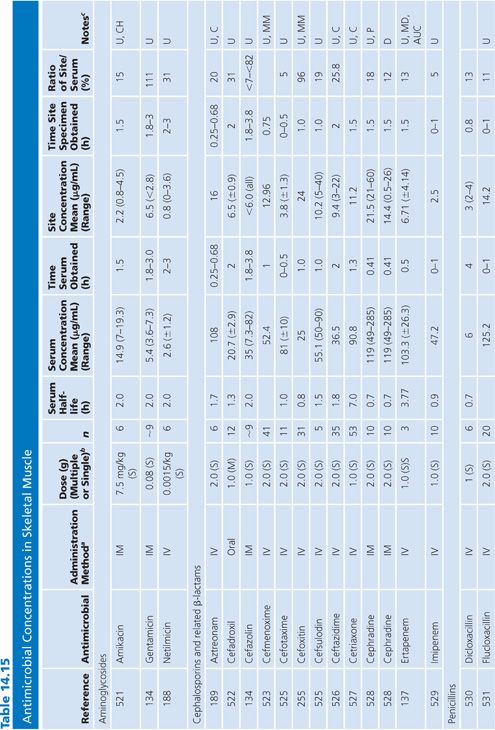
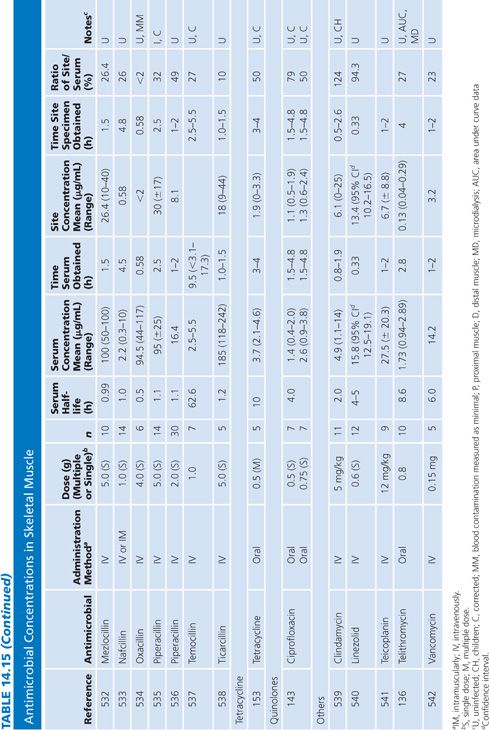
Bone
A multitude of studies on antibiotic penetration into bone have been published (Table 14.16). Most of the studies were performed on uninfected bone, following antibiotics given prophylactically to patients prior to orthopedic procedures. There is a wide variance in technique, making the results conflicting and difficult to interpret. The majority of studies reported techniques that cut or crushed the bone, suspended the material in a buffer for a variable amount of time, and then measured the antibiotic extracted into the buffer, using a microbiologic assay. Newer studies used microdialysis techniques measuring interstitial fluid concentrations within bone. Many investigators washed or rinsed the bone to remove blood after removing the sample. It is unknown whether this could also remove some of the antibiotic.
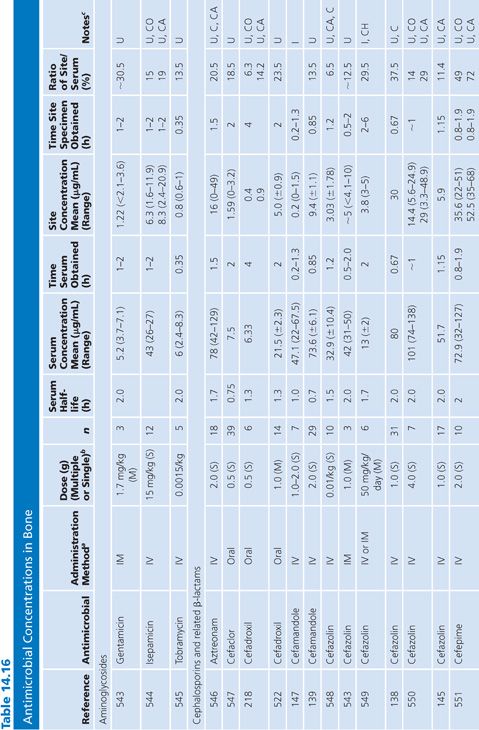
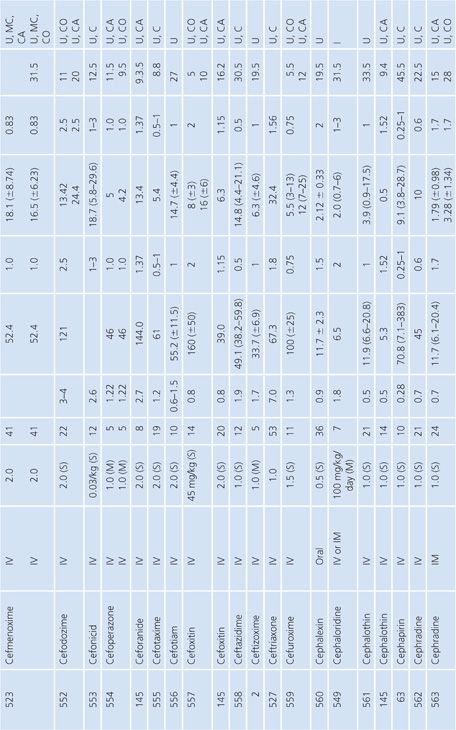
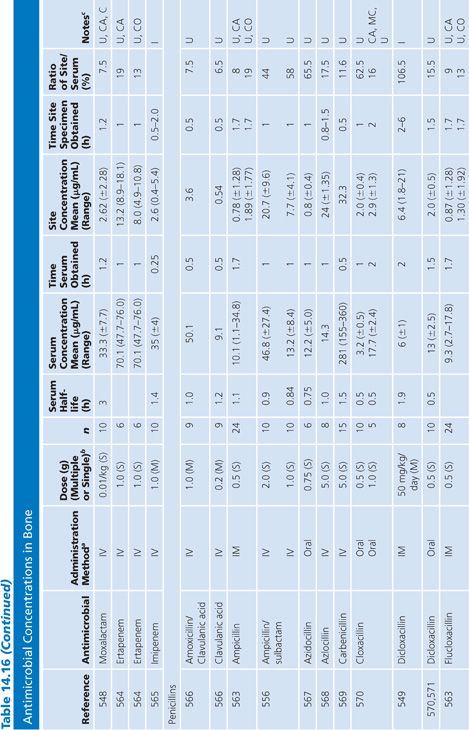
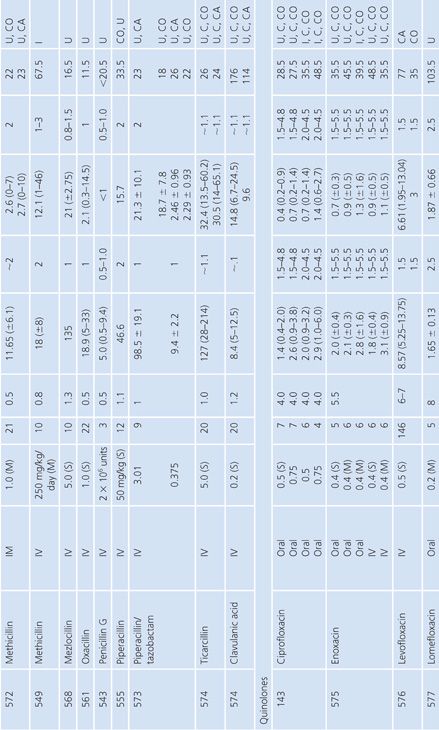
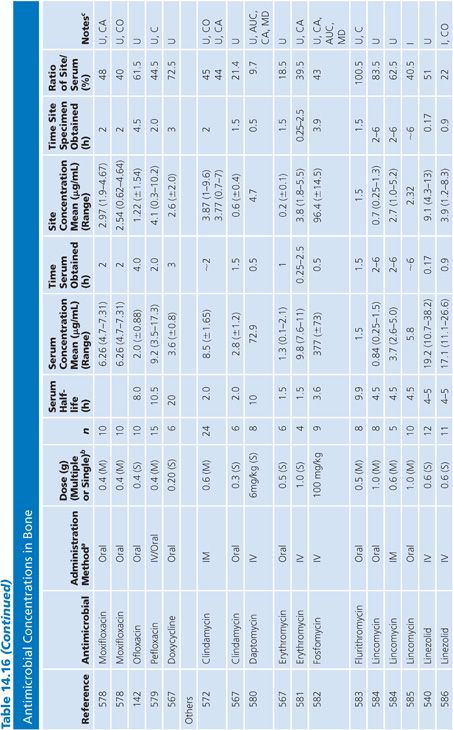
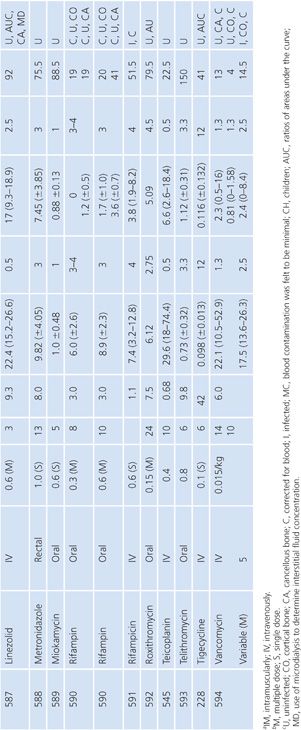
The buffer/bone mixture is usually shaken at 4°C to 6°C for 1 to 24 hours. An 8-hour shake/elution technique has resulted in 79.9% recovery of cefazolin and 77% recovery of cephradine, but only 10.8% recovery of cephalothin (138). Schurman et al. (139) found that when the extraction procedure was performed for more than 3 to 5 hours, the recovery of cephalothin, but not cefamandole, declined by 67% at 24 hours. Rosdahl et al. (140) added several antibiotics to cancellous and cortical bone specimens and then homogenized the specimens before microbiologic assay. Recovery of the antibiotic ranged from 4% to 100%. The fall in antibiotic concentration was primarily due to the instability of the antibiotics during the homogenization procedures and was not due to binding of the antibiotic to bone. Adam et al. (141) found that neither ticarcillin nor clavulanate was adsorbed to inorganic bone; however, Wittmann and Kotthaus (142) found that the hydroxyapatite portion of bone acts as a depot carrier for several quinolone antibiotics. Other investigators have found that the quinolones bind to bone samples (143) and require several extraction steps for full extraction of the antibiotic from the bone (142).
Numerous studies noted differences between cortical and cancellous bone antibiotic concentrations, with the cortical bone usually having lower drug levels. This may reflect the fact that many antibiotics do not bind to inorganic bone, or it may be due to a difference in the blood supply. Fitzgerald (144), using data compiled from animal models, concluded that there is no anatomic or physiologic barrier preventing antibiotic diffusion into bone. He concluded that, at least in the case of β-lactams and aminoglycosides, the osseous interstitial fluid concentration of an antibiotic was a reflection of the serum concentration. This agrees with the results of Williams et al. (145), who thought that, although the relationship was not clear-cut, antibiotics with high serum levels and long half-lives usually had higher bone concentrations. Hughes and Anderson (146) thought that the capillary blood supply to the bone was of critical importance in delivering the antibiotic to the bone. In one study in which necrotic bone was sampled, cefamandole concentration was usually not detectable (147). We are not aware of any conclusive studies that attempt to correlate clinical outcome with the degree of antibiotic bone penetration, either in treating osteomyelitis or in preventing postoperative infection.
Cardiac Tissue
Antibiotic levels in cardiac tissue were measured in atrial appendage, valve, or heart muscle (Table 14.17). Virtually all studies were done in uninfected hearts. Study patients received a dose of antibiotic prior to undergoing valve replacements, coronary artery bypass grafting, or repair of congenital heart abnormalities. The cephalosporins were most widely studied and showed moderate penetration into cardiac tissue, with cephalothin and cefonicid having the lowest site-to-serum ratios. Particularly high penetration into cardiac tissue was noted for clindamycin, teicoplanin, and the quinolones. Readers are cautioned to note that cardiac penetration may not correlate with the efficacy of an antibiotic in preventing infection in patients undergoing cardiac surgery.
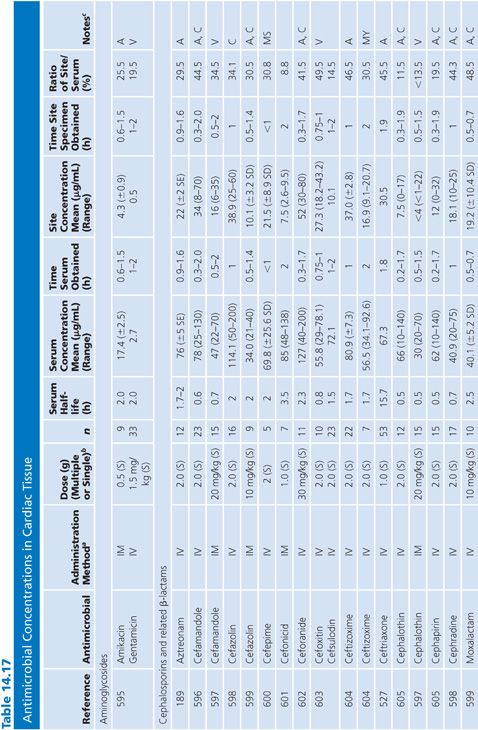
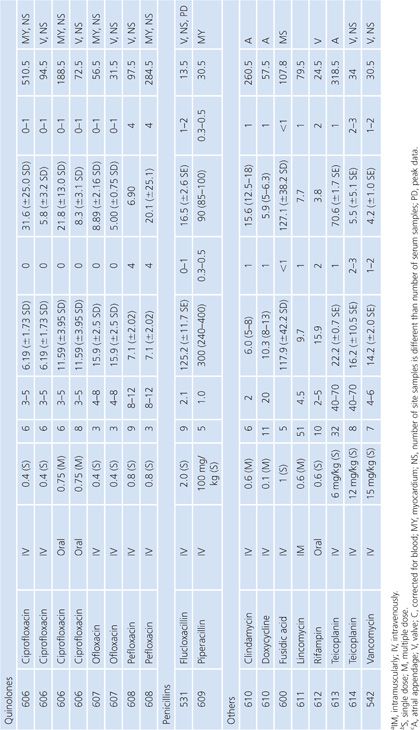
Gallbladder
Studies of antibiotic concentration in gallbladder tissue (Table 14.18) are difficult to interpret because specimens may be contaminated by bile as well as blood. Only one study cited in Table 14.18 corrected assay results for blood contamination (148). One study failed to show any difference in gallbladder levels of ceforanide when the cystic duct was obstructed or unobstructed (149). Other studies, however, revealed higher gallbladder levels of clindamycin when the common duct was patent than when it was obstructed (68) and higher levels when the gallbladder functioned than when it did not (150).
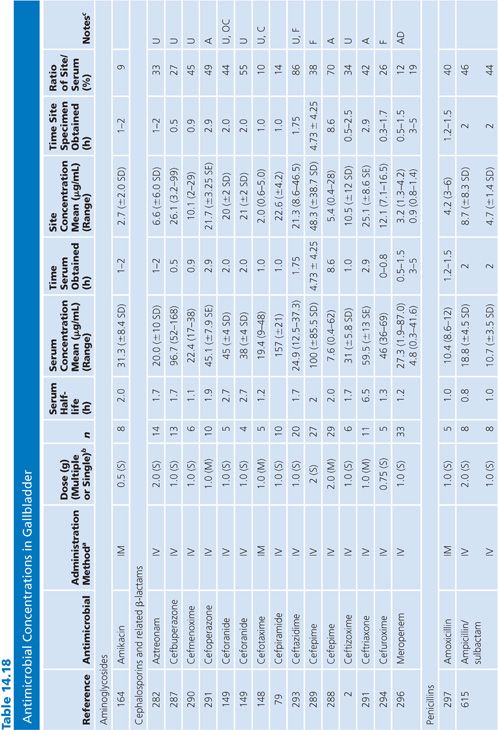
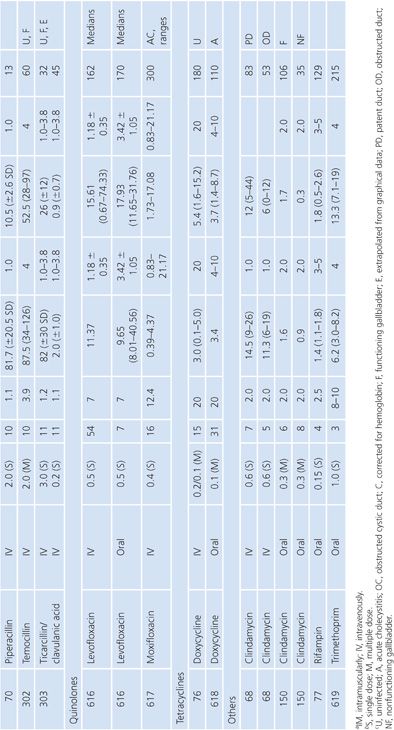
Lung Tissue
Antimicrobial concentrations in lung tissue (Table 14.19) have been measured almost exclusively following pulmonary resection of lung cancers. An exception involved a unique method of positron emission labeling of erythromycin followed by scanning of the lungs, while increasing numbers of samples of pulmonary tissue were obtained by transbronchial biopsy (151). Correction for blood contamination of specimens was done in only a few studies (151–155). The antimicrobial concentrations achieved in lung tissue were considerably higher than those in sputum (Table 14.8), but far fewer studies of lung tissue have been performed. Several drugs, such as ciprofloxacin (156), erythromycin (157), cefotaxime (158), and trimethoprim (159), showed much higher levels in lung than in blood. These studies were usually done 3 to 15 hours after the last doses of drug, at a time of relatively low serum levels, but nonetheless, they showed considerably more activity in lung tissue than in serum. Many of these agents (ciprofloxacin, erythromycin, and rifampin) are concentrated within cells, which may explain their high tissue levels. A few investigators have examined the concentrations of drug in infected or inflamed lung tissue (151,159–161). These levels were not substantially different from those in normal lung tissue from the same individuals. We are unaware of good correlation between lung antimicrobial concentrations and clinical therapeutic or prophylactic efficacy in humans. Therefore, clinical inferences from these data should be made with caution.
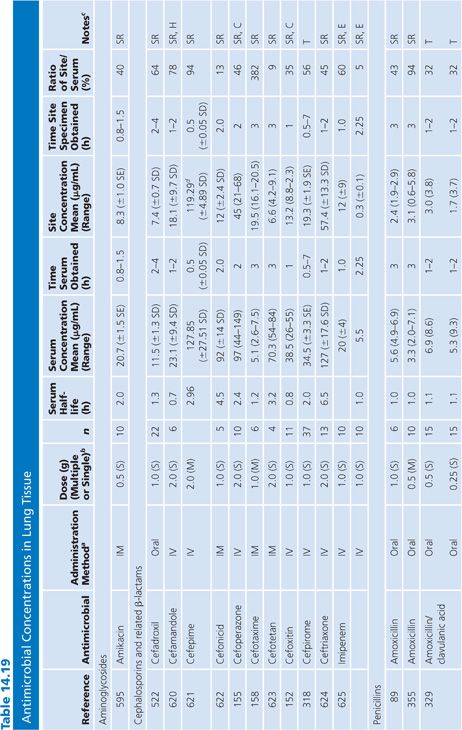
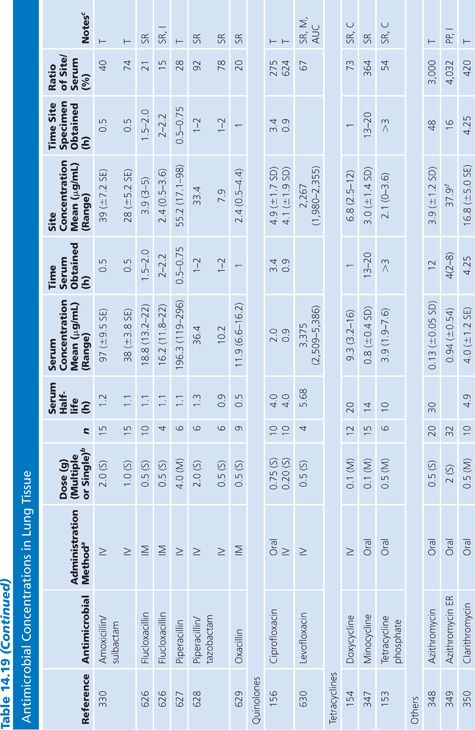
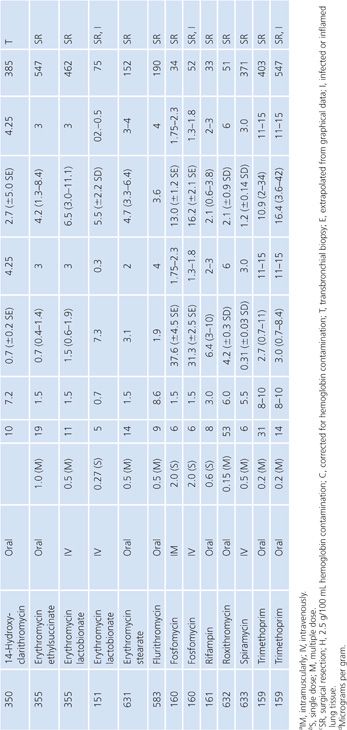
Prostatic Tissue
Numerous studies have measured antimicrobial concentrations in human prostatic tissue (Table 14.20). Most of the studies were performed with patients without infection, undergoing transurethral or suprapubic prostatectomy. Techniques for tissue preparation were variable. As is the case with prostatic secretions, the prostatic tissue concentrations observed in most of these studies may be due to urinary contamination and therefore must be interpreted with caution (107,162).
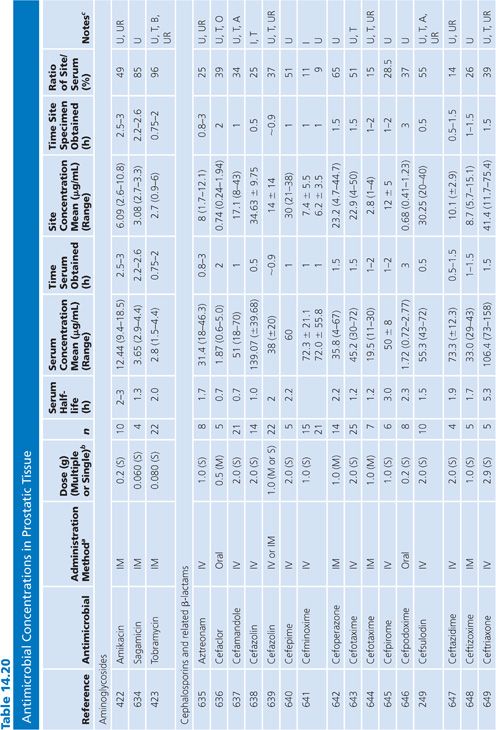
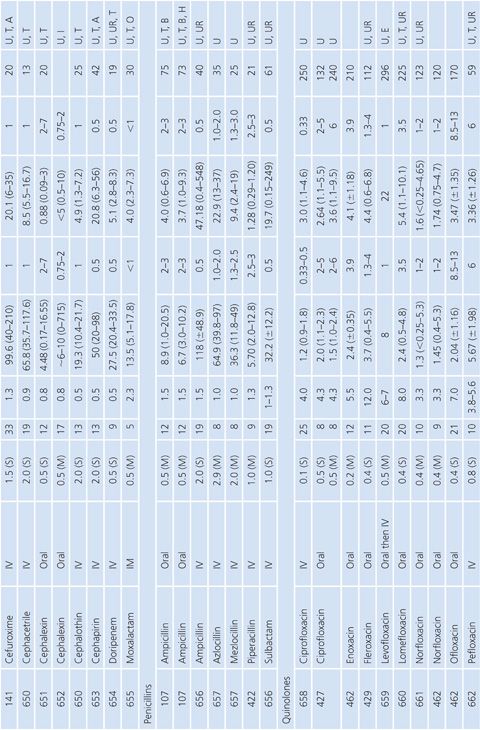
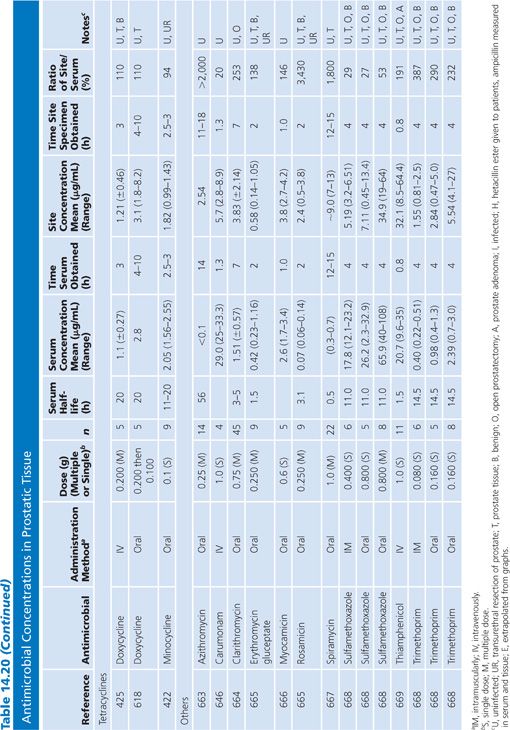
INTERPRETATION OF SERUM CONCENTRATIONS OF ANTIMICROBIALS
Interpretation of antimicrobial serum or plasma concentration data requires a basic understanding of the principles that govern pharmacokinetics: absorption, distribution, metabolism, and excretion of drug. In addition, method of administration, number of dosage administrations prior to sample collection, and timing of the sample collection with respect to the previous dose are equally important factors to consider when comparing data (163–165). Figure 14.2 depicts theoretical serum concentration data, using a two-compartment open simulation model, following a single 1-g dose of an antimicrobial by four different methods: intravenous bolus (1-minute infusion), 30-minute intravenous infusion, oral, and intramuscular administration. The apparent volume of distribution, bioavailability (100%), rate of elimination, and transfer rate constants between the central and peripheral compartments were all held constant in order to show just how different theoretical concentration-time curves may look when only one of these variables (administration) is altered.
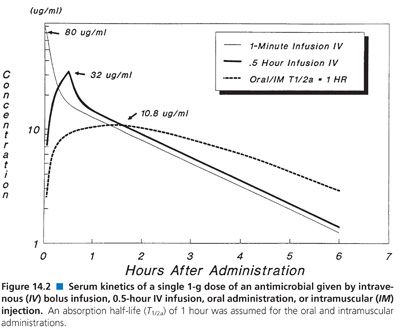
Absorption
Absorption is the process by which antibiotic transfers from the site of administration (such as intravenous, intramuscular, oral, and topical) into the general circulation (central compartment) of the body, and it ranges from 0% to 100%. Percentage bioavailability (Table 14.21) is simply 100 times the ratio of the amount of active antibiotic (A) reaching the general circulation to the amount administered (dose).
Percentage bioavailability = 100 × A/dose
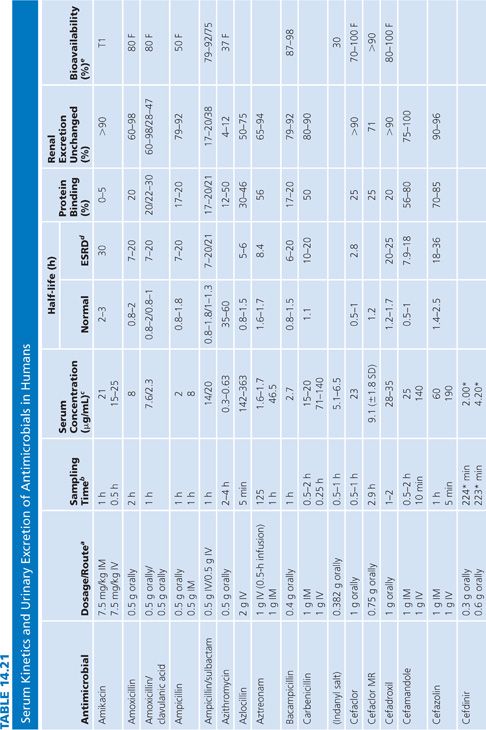
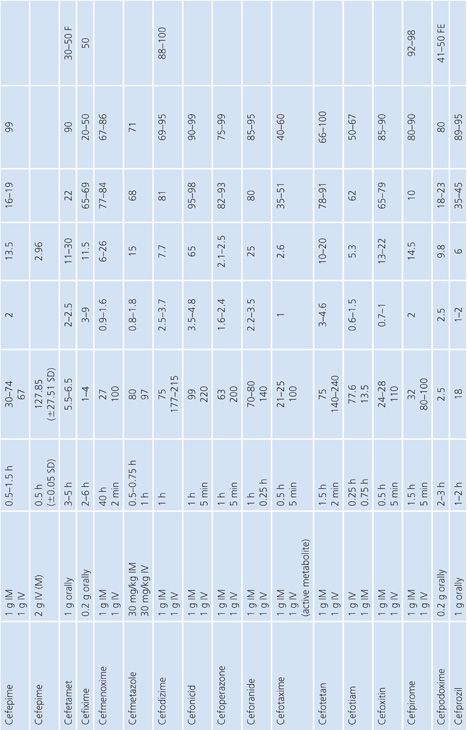
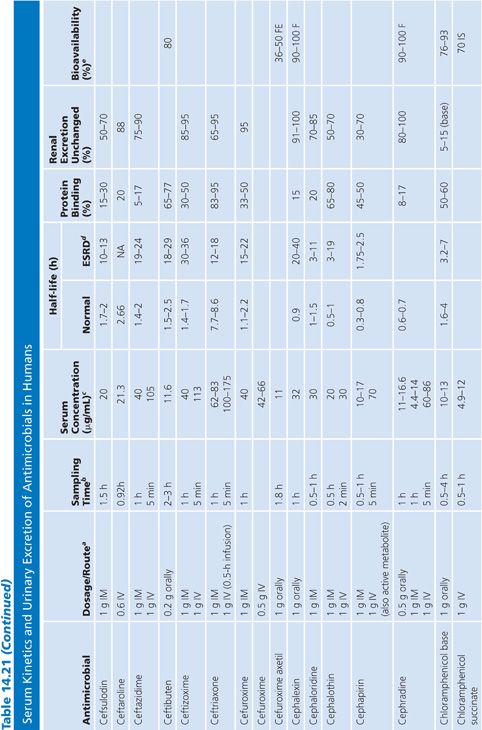
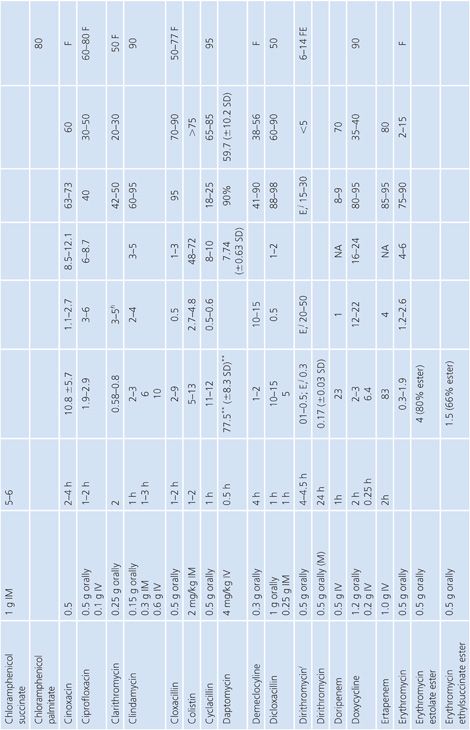
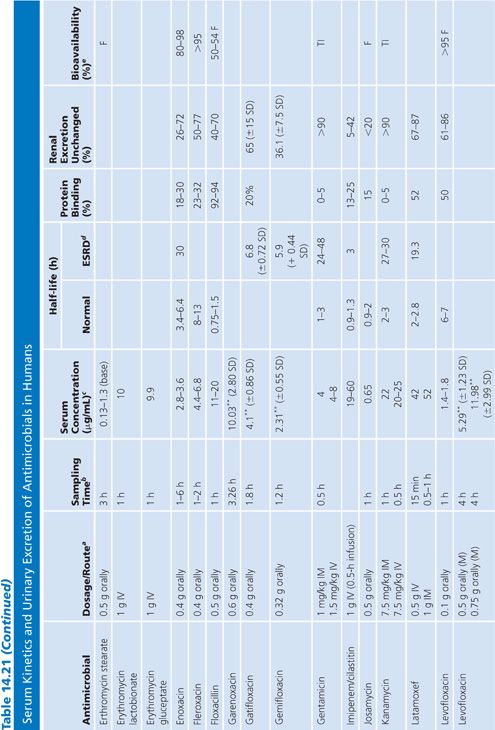
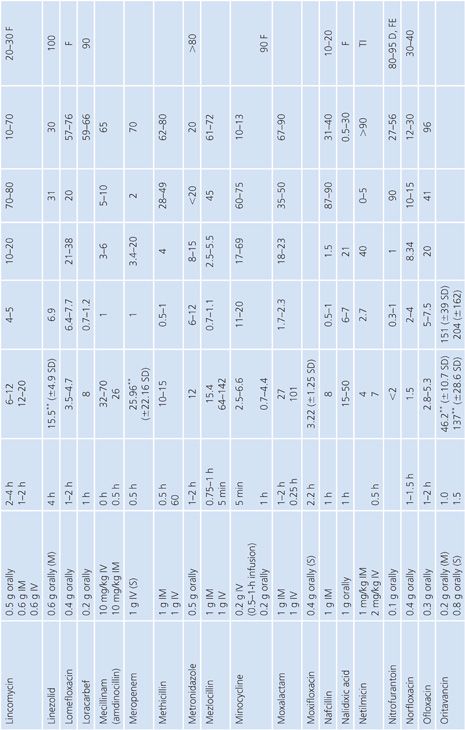
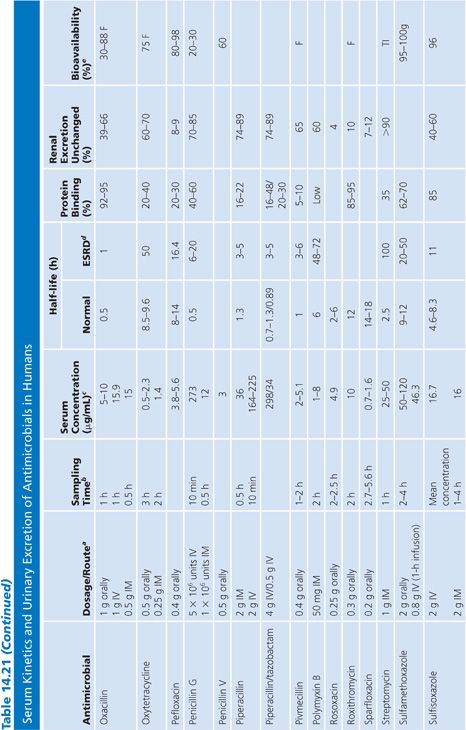
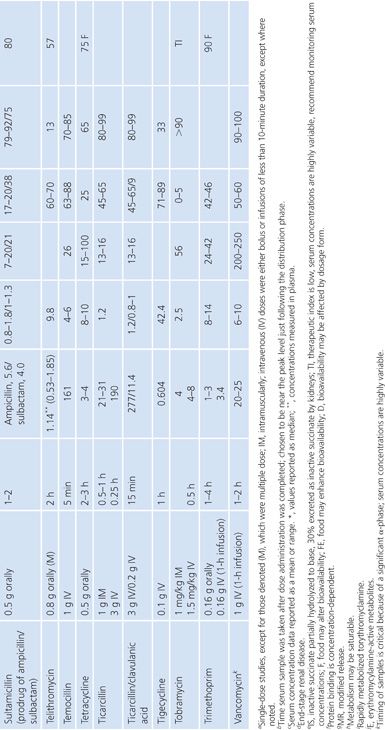
For most antimicrobials administered intravenously or intramuscularly, percentage bioavailability is 100%. However, some, such as chloramphenicol succinate, are in an inactive form when given parenterally and the actual percentage bioavailability may be less than 100% because of partial conversion. Absorption from oral administration is less than 100% for most antimicrobials; therefore, the area under the serum concentration–time curve following oral administration is less than that following parenteral administration, with all other variables held constant. Percentage bioavailability refers only to the extent of absorption; it gives no indication of how rapidly the antimicrobial is absorbed and does not account for protein binding.
Rate of Administration
Difficulty in interpreting differences in concentration with various routes of administration is often attributed to the respective rates of antimicrobial administration. Generally, intravenous administration is achieved through a zero-order process (constant rate of administration over time) and intramuscular and oral administrations are thought to follow a first-order process (Fick’s law of passive diffusion). Intramuscular and oral absorption rates are also influenced by a number of local factors, such as pH, gastrointestinal motility, dosage form, food, and muscle perfusion, to mention a few; each of which may be significant. As a result, peak serum concentration following intramuscular or oral administration tends to be lower and less predictable than that after intravenous administration. Again, the simulations in Figure 14.2, in which the oral and intramuscular half-life of drug absorption (T1/2a) was held constant (1 hour) and the intravenous rates differ, show the effects observed by simply changing the rate of antibiotic administration.
Distribution
Once the antibiotic reaches the general circulation (central compartment), the antibiotic begins to diffuse into other body spaces and tissues (peripheral compartments) not at equilibrium with the central compartment (Fig. 14.1). This process is often referred to as distribution, and the time period during which this is most evident on the serum concentration–time curve is often referred to as the distribution phase or α-phase. Although distribution takes place following all forms of antimicrobial administration, the distribution phase is most apparent immediately following intravenous bolus or short infusion, which makes timing of sample collection most critical for concentration comparisons. The distribution phase of the serum concentration–time curve is generally short-lived (less than 1 hour); however, minor differences in the time of serum sample collection during the distribution phase may result in major differences in antimicrobial concentrations and therefore interpretation. Because of the difficulty in timing and collecting samples in and around the distribution phase of the curve, peak serum concentration data reported in the literature are often conflicting and misleading. The distribution phase for most antibiotics is of brief duration and is difficult to characterize. In order to avoid confusion and subsequent misinterpretation, many authors choose not to sample during the distribution phase but rather report the time at which serum samples for determination of antibiotic concentration were obtained following antibiotic administration. Data on achievable serum concentrations in Table 14.21 are presented with the time at which samples were obtained. In general, intravenous administration results in serum concentrations that are greater than those following oral or intramuscular administration and are most vulnerable to misinterpretation due to the distribution phase.
Elimination
Elimination is the loss of active antibiotic from the body and is due to excretion and/or metabolism. Excretion is the loss of unchanged antibiotic, and metabolism is the loss due to conversion of the antibiotic to another chemical compound (metabolite). Metabolites may or may not have antimicrobial activity. For most antibiotics, elimination is a first-order process, which simply means that serum concentration declines exponentially with time:
Ct = C × e−kel × t
where C is serum concentration, Ct is the serum concentration at some time t after C was determined, and kef is an elimination rate constant. With first-order elimination, the time for the serum concentration to decline by one-half (half-life) is constant:
t1/2 = (ln2)/kel
where t1/2 is the serum half-life and kef is the elimination rate constant. Knowing the serum half-life, one can then estimate the fraction of the dose that remains in the body at any time after the dose was given:
Fraction remaining = e−0.693n
where n is the number of half-lives that have elapsed since the dose was administered.
Renal Excretion
Most antimicrobials are eliminated in the urine, either unchanged or as metabolites. The data on achievable urinary concentration are often difficult to interpret. Urinary concentrations are constantly changing as a function of changing serum concentration and urine volume. The fraction of drug in general circulation that is excreted unchanged in the urine, fe, is a pharmacokinetic parameter that describes the contribution of urinary elimination to the overall elimination of antibiotic from the body.
fe = total drug excreted unchanged/total dose
The percentage of antibiotic excreted unchanged (fe 100) is high for antibiotics with renal excretion as the sole or primary route of elimination and low for antibiotics that are not readily excreted in the urine or that undergo significant metabolism. Determination of fe requires both an assay that is specific for the parent compound and a sampling time sufficiently long to ensure complete urinary excretion (at least five times the half-life).
Appendix 14.1 shows urinary excretion, metabolites, and reported urine levels for some selected antimicrobial agents.
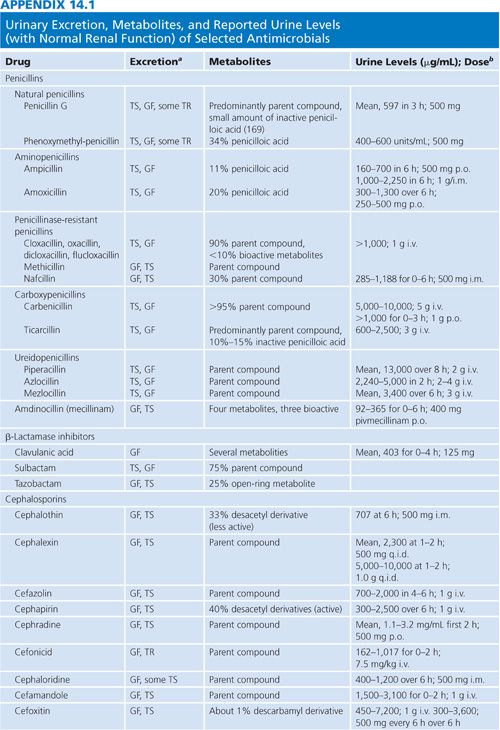
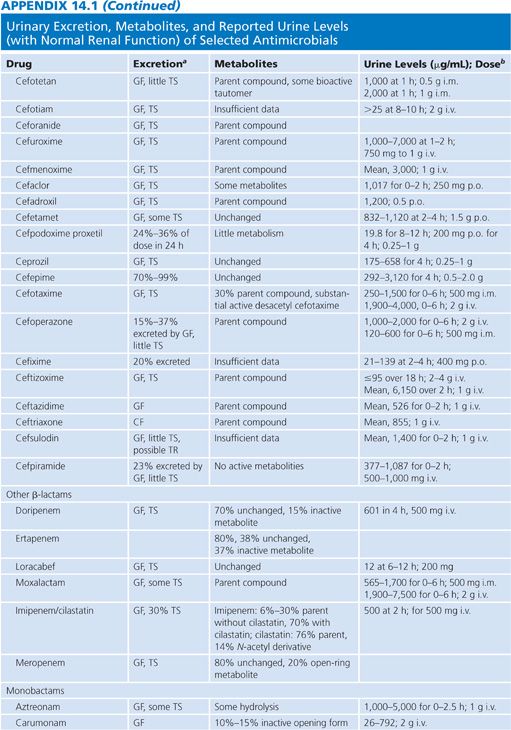
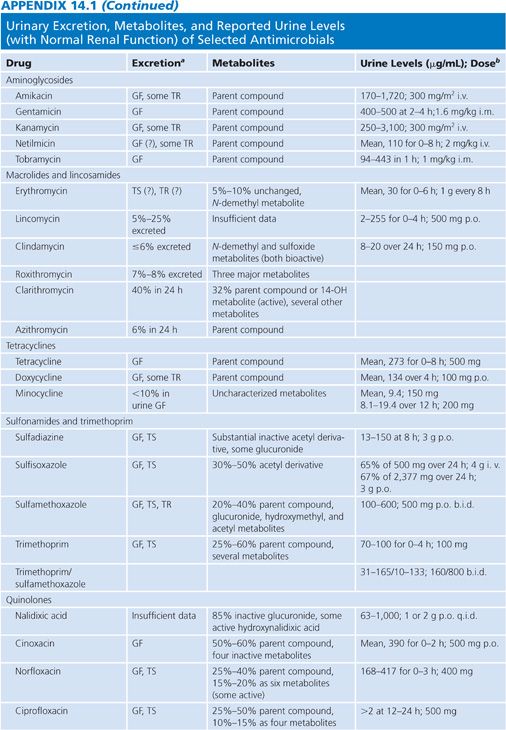
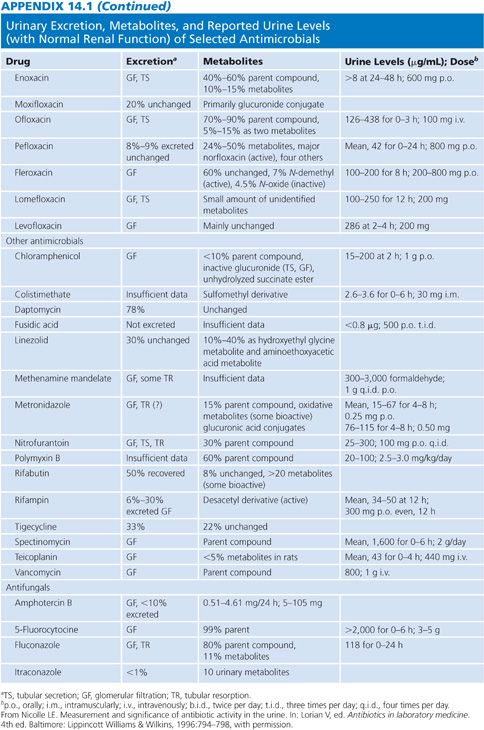
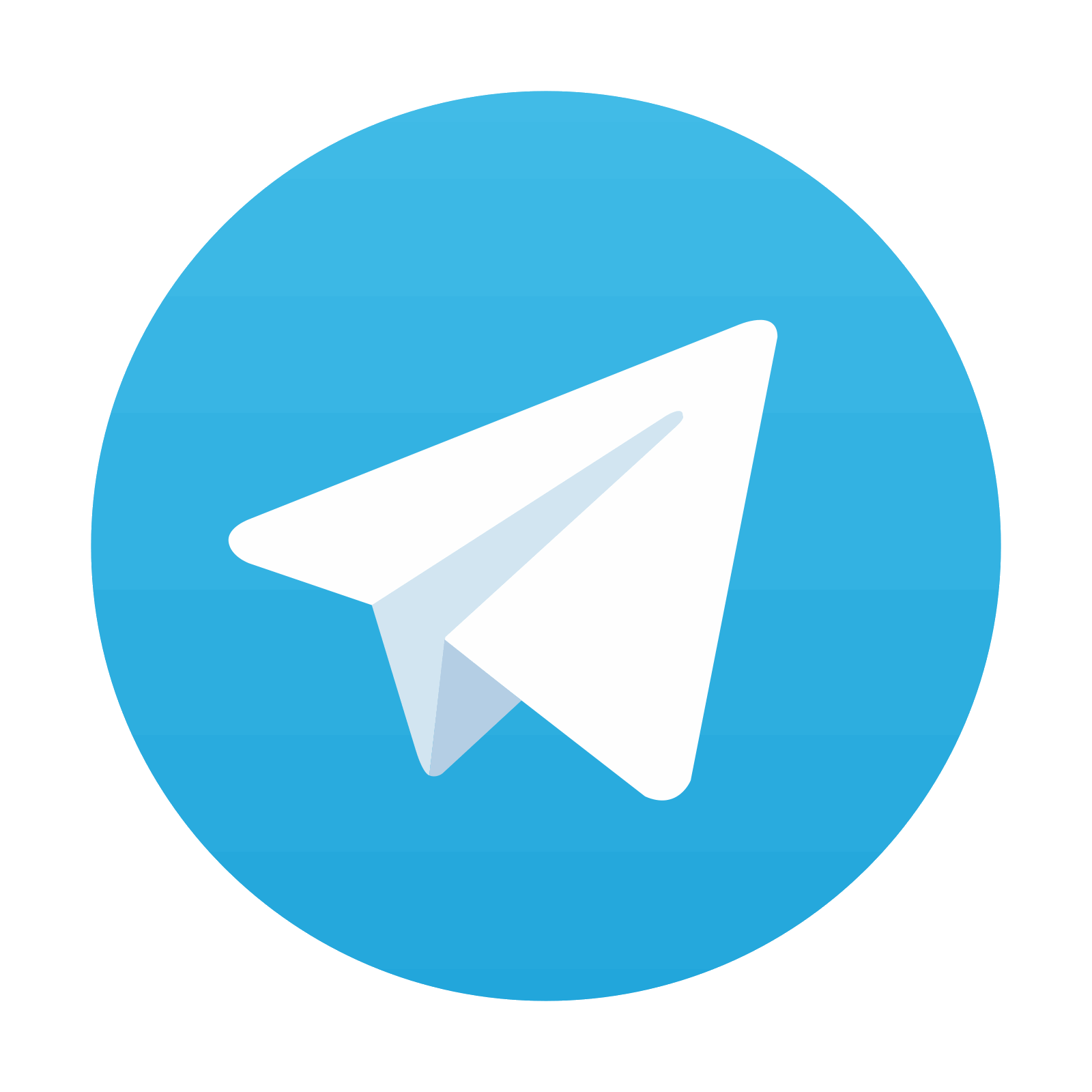
Stay updated, free articles. Join our Telegram channel
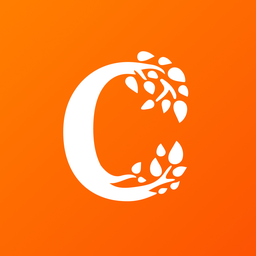
Full access? Get Clinical Tree
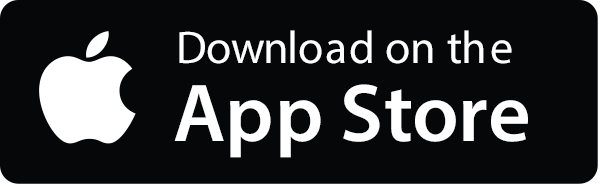
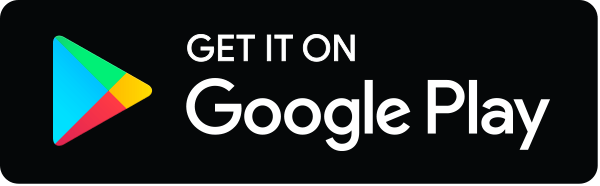