OBJECTIVES
After studying this chapter, you should be able to:
Name the various types of glia and their functions.
Name the parts of a neuron and their functions.
Describe the chemical nature of myelin, and summarize the differences in the ways in which unmyelinated and myelinated neurons conduct impulses.
Describe orthograde and retrograde axonal transport.
Describe the changes in ionic channels that underlie the action potential.
List the various nerve fiber types found in the mammalian nervous system.
Describe the function of neurotrophins.
INTRODUCTION
The human central nervous system (CNS) contains about 1011 (100 billion) neurons. It also contains 2–10 times this number of glial cells. The CNS is a complex organ; it has been calculated that 40% of the human genes participate, at least to a degree, in its formation. The neurons, the basic building blocks of the nervous system, have evolved from primitive neuroeffector cells that respond to various stimuli by contracting. In more complex animals, contraction has become the specialized function of muscle cells, whereas integration and transmission of nerve impulses have become the specialized functions of neurons. Neurons and glial cells along with brain capillaries form a functional unit that is required for normal brain function, including synaptic activity, extracellular fluid homeostasis, energy metabolism, and neural protection. Disturbances in the interaction of these elements are the pathophysiological basis for many neurologic disorders (eg, cerebral ischemia, seizures, neurodegenerative diseases, and cerebral edema). This chapter describes the cellular components of the CNS and the excitability of neurons, which involves the genesis of electrical signals that enable neurons to integrate and transmit impulses (eg, action potentials, receptor potentials, and synaptic potentials).
CELLULAR ELEMENTS IN THE CNS
For many years following their discovery, glial cells (or glia) were viewed as CNS connective tissue. In fact, the word glia is Greek for glue. However, today these cells are recognized for their role in communication within the CNS in partnership with neurons. Unlike neurons, glial cells continue to undergo cell division in adulthood and their ability to proliferate is particularly noticeable after brain injury (eg, stroke).
There are two major types of glial cells in the vertebrate nervous system: microglia and macroglia. Microglia are immune system cells; they are scavenger cells that resemble tissue macrophages and remove debris resulting from injury, infection, and disease (eg, multiple sclerosis [MS], AIDS-related dementia, Parkinson disease, and Alzheimer disease). Microglia arise from macrophages outside of the nervous system and are physiologically and embryologically unrelated to other neural cell types.
There are three types of macroglia: oligodendrocytes, Schwann cells, and astrocytes (Figure 4–1). Oligodendrocytes and Schwann cells are involved in myelin formation around axons in the CNS and peripheral nervous system, respectively. Astrocytes, which are found throughout the brain, are further subdivided into two groups. Fibrous astrocytes, which contain many intermediate filaments, are found primarily in white matter. Protoplasmic astrocytes are found in gray matter and have a granular cytoplasm. Both types of astrocytes send processes to blood vessels, where they induce capillaries to form the tight junctions making up the blood–brain barrier. They also send processes that envelop synapses and the surface of nerve cells. Protoplasmic astrocytes have a membrane potential that varies with the external K+ concentration but do not generate propagated potentials. They produce substances that are tropic to neurons, and they help maintain the appropriate concentration of ions and neurotransmitters by taking up K+ and the neurotransmitters glutamate and γ-aminobutyrate (GABA).
FIGURE 4–1
The principal types of macroglia in the nervous system. A) Oligodendrocytes are small with relatively few processes. Those in the white matter provide myelin, and those in the gray matter support neurons. B) Schwann cells provide myelin to the peripheral nervous system. Each cell forms a segment of myelin sheath about 1 mm long; the sheath assumes its form as the inner tongue of the Schwann cell turns around the axon several times, wrapping in concentric layers. Intervals between segments of myelin are the nodes of Ranvier. C) Astrocytes are the most common glia in the CNS and are characterized by their starlike shape. They contact both capillaries and neurons and are thought to have a nutritive function. They are also involved in forming the blood–brain barrier. (Reproduced with permission from Kandel ER, Schwartz JH, Jessell TM, Siegelbaum SA, Hudspeth AJ (editors): Principles of Neural Science, 5th ed. New York, NY: McGraw-Hill; 2013.)
Neurons in the mammalian CNS come in many different shapes and sizes. Most have the same parts as the typical spinal motor neuron illustrated in Figure 4–2. The cell body (soma) contains the nucleus and is the metabolic center of the neuron. Neurons have several processes called dendrites that extend outward from the cell body and arborize extensively. Particularly in the cerebral and cerebellar cortex, the dendrites have small knobby projections called dendritic spines. A typical neuron also has a long fibrous axon that originates from a somewhat thickened area of the cell body, the axon hillock. The first portion of the axon is called the initial segment. The axon divides into presynaptic terminals, each ending in a number of synaptic knobs that are also called terminal buttons or boutons. They contain granules or vesicles in which the synaptic transmitters secreted by the nerves are stored. Based on the number of processes that emanate from their cell body, neurons can be classified as unipolar, bipolar, pseudounipolar, and multipolar (Figure 4–3).
FIGURE 4–2
Motor neuron with a myelinated axon. A motor neuron is composed of a cell body (soma) with a nucleus, several processes called dendrites, and a long fibrous axon that originates from the axon hillock. The first portion of the axon is called the initial segment. A myelin sheath forms from Schwann cells and surrounds the axon except at its ending and at the nodes of Ranvier. Terminal buttons (boutons) are located at the terminal endings.
FIGURE 4–3
Some of the types of neurons in the mammalian nervous system. A) Unipolar neurons have one process, with different segments serving as receptive surfaces and releasing terminals. B) Bipolar neurons have two specialized processes: a dendrite that carries information to the cell and an axon that transmits information from the cell. C) Some sensory neurons are in a subclass of bipolar cells called pseudounipolar cells. As the cell develops, a single process splits into two, both of which function as axons—one going to skin or muscle and another to the spinal cord. D) Multipolar cells have one axon and many dendrites. Examples include motor neurons, hippocampal pyramidal cells with dendrites in the apex and base, and cerebellar Purkinje cells with an extensive dendritic tree in a single plane. (Reproduced with permission from Kandel ER, Schwartz JH, Jessell TM, Siegelbaum SA, Hudspeth AJ (editors): Principles of Neural Science, 5th ed. New York, NY: McGraw-Hill; 2013.)
The conventional terminology used for the parts of a neuron works well enough for spinal motor neurons and interneurons, but there are problems in terms of “dendrites” and “axons” when it is applied to other types of neurons found in the nervous system. From a functional point of view, neurons generally have four important zones: (1) a receptor, or dendritic zone, where multiple local potential changes generated by synaptic connections are integrated; (2) a site where propagated action potentials are generated (the initial segment in spinal motor neurons, the initial node of Ranvier in cutaneous sensory neurons); (3) an axonal process that transmits propagated impulses to the nerve endings; and (4) the nerve endings, where action potentials cause the release of synaptic transmitters. The cell body is often located at the dendritic zone end of the axon, but it can be within the axon (eg, auditory neurons) or attached to the side of the axon (eg, cutaneous neurons). Its location makes no difference as far as the receptor function of the dendritic zone and the transmission function of the axon are concerned.
The axons of many neurons are myelinated, that is, they acquire a sheath of myelin, a protein–lipid complex that is wrapped around the axon (Figure 4–1B). In the peripheral nervous system, myelin forms when a Schwann cell wraps its membrane around an axon up to 100 times. The myelin is then compacted when the extracellular portions of a membrane protein called protein zero (P0) lock to the extracellular portions of P0 in the apposing membrane. Various mutations in the gene for P0 cause peripheral neuropathies; 29 different mutations have been described that cause symptoms ranging from mild to severe. The myelin sheath envelops the axon except at its ending and at the nodes of Ranvier, periodic 1-μm constrictions that are about 1 mm apart (Figure 4–2). The insulating function of myelin is discussed later in this chapter. Not all neurons are myelinated; some are unmyelinated, that is, simply surrounded by Schwann cells without the wrapping of the Schwann cell membrane that produces myelin around the axon.
In the CNS of mammals, most neurons are myelinated, but the cells that form the myelin are oligodendrocytes rather than Schwann cells (Figure 4–1). Unlike the Schwann cell, which forms the myelin between two nodes of Ranvier on a single neuron, oligodendrocytes emit multiple processes that form myelin on many neighboring axons. In MS, patchy destruction of myelin occurs in the CNS (Clinical Box 4–1). The loss of myelin is associated with delayed or blocked conduction in the demyelinated axons.
CLINICAL BOX 4–1 Demyelinating Diseases
Normal conduction of action potentials relies on the insulating properties of myelin. Thus, defects in myelin can have major adverse neurologic consequences. One example is multiple sclerosis (MS), an autoimmune disease that affects over 3 million people worldwide, usually striking between the ages of 20 and 50 and affecting women about twice as often as men. The cause of MS appears to include both genetic and environmental factors. It is most common among whites living in countries with temperate climates including Europe, southern Canada, northern United States, and southeastern Australia. Environmental triggers include early exposure to viruses such as Epstein-Barr virus and those that cause measles, herpes, chickenpox, or influenza. In MS, antibodies and white blood cells in the immune system attack myelin, causing inflammation and injury to the sheath and eventually the nerves that it surrounds. Loss of myelin leads to leakage of K+ through voltage-gated channels, hyperpolarization, and failure to conduct action potentials. Initial presentation commonly includes reports of paraparesis (weakness in lower extremities) that may be accompanied by mild spasticity and hyperreflexia; paresthesia; numbness; urinary incontinence; and heat intolerance. Clinical assessment often reports optic neuritis, characterized by blurred vision, a change in color perception, visual field defect (central scotoma), and pain with eye movements; dysarthria; and dysphagia. Symptoms are often exacerbated by increased body temperature or ambient temperature. Progression of the disease is quite variable. In the most common form called relapsing-remitting MS, transient episodes appear suddenly, last a few weeks or months, and then gradually disappear. Subsequent episodes can appear years later, and eventually full recovery does not occur. A steadily worsening course with only minor periods of remission (secondary-progressive MS) develops later in many individuals. Others have a progressive form of the disease in which there are no periods of remission (primary-progressive MS). Diagnosing MS is very difficult and generally is delayed until multiple episodes occur with deficits separated in time and space. Nerve conduction tests can detect slowed conduction in motor and sensory pathways. Cerebral spinal fluid analysis can detect the presence of oligoclonal bands indicative of an abnormal immune reaction against myelin. The most definitive assessment is magnetic resonance imaging (MRI) to visualize multiple scarred (sclerotic) areas or plaques in the brain. These plaques often appear in the periventricular regions of the cerebral hemispheres.
THERAPEUTIC HIGHLIGHTSAlthough there is no cure for MS, corticosteroids (eg, prednisone) are the most common treatment used to reduce the inflammation that is accentuated during a relapse. Some drug treatments are designed to modify the course of the disease. For example, daily injections of β-interferons suppress the immune response to reduce the severity and slow the progression of the disease. Glatiramer acetate may block the immune system’s attack on the myelin. Natalizumab interferes with the ability of potentially damaging immune cells to move from the bloodstream to the CNS. A clinical trial using B cell–depleting therapy with rituximab, an anti-CD20 monoclonal antibody, showed that the progression of the disease was slowed in patients younger than 51 years in whom the primary-progressive form of MS was diagnosed. Another clinical trial has shown that oral administration of fingolimod slowed the progression of the relapsing-remitting form of MS. This immunosuppressive drug acts by sequestering lymphocytes in the lymph nodes, thereby limiting their access to the CNS.
AXONAL TRANSPORT
Neurons are secretory cells, but they differ from other secretory cells in that the secretory zone is generally at the end of the axon, far removed from the cell body. The apparatus for protein synthesis is located for the most part in the cell body, with transport of proteins and polypeptides to the axonal ending by axoplasmic flow. Thus, the cell body maintains the functional and anatomic integrity of the axon; if the axon is cut, the part distal to the cut degenerates (Wallerian degeneration).
Orthograde transport occurs along microtubules that run along the length of the axon and requires two molecular motors, dynein and kinesin (Figure 4–4). Orthograde transport moves from the cell body toward the axon terminals. It has both fast and slow components, fast axonal transport occurs at about 400 mm/day, and slow axonal transport occurs at 0.5 to 10 mm/day. Retrograde transport, which is in the opposite direction (from the nerve ending to the cell body), occurs along microtubules at about 200 mm/day. Synaptic vesicles recycle in the membrane, but some used vesicles are carried back to the cell body and deposited in lysosomes. Some materials taken up at the ending by endocytosis, including nerve growth factor (NGF) and some viruses, are also transported back to the cell body. A potentially important exception to these principles seems to occur in some dendrites. In them, single strands of mRNA transported from the cell body make contact with appropriate ribosomes, and protein synthesis appears to create local protein domains.
FIGURE 4–4
Axonal transport along microtubules by dynein and kinesin. Fast (400 mm/day) and slow (0.5–10 mm/day) axonal orthograde transport occurs along microtubules that run along the length of the axon from the cell body to the terminal. Retrograde transport (200 mm/day) occurs from the terminal to the cell body. (Reproduced with permission from Widmaier EP, Raff H, Strang KT: Vander’s Human Physiology. New York, NY: McGraw-Hill; 2008.)
EXCITATION & CONDUCTION
A hallmark of nerve cells is their excitable membrane. Nerve cells respond to electrical, chemical, or mechanical stimuli. Two types of physicochemical disturbances are produced: local, nonpropagated potentials called, depending on their location, synaptic, generator, or electrotonic potentials; and propagated potentials, the action potentials (or nerve impulses). Action potentials are the primary electrical responses of neurons and other excitable tissues, and they are the main form of communication within the nervous system. They are due to changes in the conduction of ions across the cell membrane. The electrical events in neurons are rapid, being measured in milliseconds (ms); and the potential changes are small, being measured in millivolts (mV).
The impulse is normally transmitted (conducted) along the axon to its termination. Nerves are not “telephone wires” that transmit impulses passively; conduction of nerve impulses, although rapid, is much slower than that of electricity. Nerve tissue is in fact a relatively poor passive conductor, and it would take a potential of many volts to produce a signal of a fraction of a volt at the other end of a meter-long axon in the absence of active processes in the nerve. Instead, conduction is an active, self-propagating process, and the impulse moves along the nerve at a constant amplitude and velocity. The process is often compared to what happens when a match is applied to one end of a trail of gunpowder; by igniting the powder particles immediately in front of it, the flame moves steadily down the trail to its end as it is extinguished in its wake.
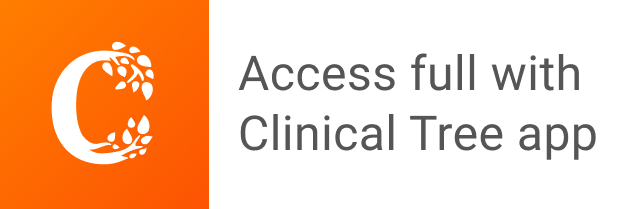