OBJECTIVES
After studying this chapter, you should be able to:
Differentiate the major classes of muscle in the body.
Describe the molecular and electrical makeup of muscle cell excitation–contraction coupling.
Define elements of the sarcomere that underlie striated muscle contraction.
Differentiate the role(s) for Ca2+ in skeletal, cardiac, and smooth muscle contraction.
Appreciate muscle cell diversity and function.
INTRODUCTION
Muscle cells, like neurons, can be excited chemically, electrically, and mechanically to produce an action potential that is transmitted along their cell membranes. Unlike neurons, they respond to stimuli by activating a contractile mechanism. The contractile protein myosin and the cytoskeletal protein actin are abundant in muscle, where they are the primary structural components that bring about contraction.
Muscle is generally divided into three types: skeletal, cardiac, and smooth, although smooth muscle is not a homogeneous single category. Skeletal muscle makes up the great mass of the somatic musculature. It has well-developed cross-striations, does not normally contract in the absence of nervous stimulation, lacks anatomic and functional connections between individual muscle fibers, and is generally under voluntary control. Cardiac muscle also has cross-striations, but it is functionally syncytial and, although it can be modulated via the autonomic nervous system, it can contract rhythmically in the absence of external innervation owing to the presence in the myocardium of pacemaker cells that discharge spontaneously (see Chapter 29). Smooth muscle lacks cross-striations and can be further subdivided into two broad types: unitary (or visceral) smooth muscle and multiunit smooth muscle. The type found in most hollow viscera is functionally syncytial and contains pacemakers that discharge irregularly. The multiunit type found in the eye and in some other locations is not spontaneously active and resembles skeletal muscle in graded contractile ability.
SKELETAL MUSCLE MORPHOLOGY
Skeletal muscle is made up of individual muscle fibers that are the “building blocks” of the muscular system in the same sense that the neurons are the building blocks of the nervous system. Most skeletal muscles begin and end in tendons, and the muscle fibers are arranged in parallel between the tendinous ends, so that the force of contraction of the units is additive. Each muscle fiber is a single cell that is multinucleated, long, cylindrical, and surrounded by a cell membrane, the sarcolemma (Figure 5–1). There are no syncytial bridges between cells. The muscle fibers are made up of myofibrils, which are divisible into individual filaments. These myofilaments contain several proteins that together make up the contractile machinery of the skeletal muscle.
FIGURE 5–1
Mammalian skeletal muscle. A) A single muscle fiber surrounded by its sarcolemma has been cut away to show individual myofibrils. The cut surface of the myofibrils shows the arrays of thick and thin filaments. The sarcoplasmic reticulum with its transverse (T) tubules and terminal cisterns surrounds each myofibril. The T tubules invaginate from the sarcolemma and contact the myofibrils twice in every sarcomere. Mitochondria are found between the myofibrils and a basal lamina surrounds the sarcolemma. B and C) Structural elements of myofibril shown in detail (see also Figure 5-2).
The contractile mechanism in skeletal muscle largely depends on the proteins myosin-II, actin, tropomyosin, and troponin. Troponin is made up of three subunits: troponin I, troponin T, and troponin C. Other important proteins in muscle are involved in maintaining the proteins that participate in contraction in appropriate structural relation to one another and to the extracellular matrix.
Differences in the refractive indexes of the various parts of the muscle fiber are responsible for the characteristic cross-striations seen in skeletal muscle when viewed under the microscope. The parts of the cross-striations are frequently identified by letters (Figure 5–2). The light I band is divided by the dark Z line, and the dark A band has the lighter H band in its center. A transverse M line is seen in the middle of the H band, and this line plus the narrow light areas on either side of it are sometimes called the pseudo-H zone. The area between two adjacent Z lines is called a sarcomere. The orderly arrangement of actin, myosin, and related proteins that produces this pattern can also be seen in Figures 5–1, 5–2. The thick filaments, which are about twice the diameter of the thin filaments, are made up of myosin; the thin filaments are made up of actin, tropomyosin, and troponin. The thick filaments are lined up to form the A bands, whereas the array of thin filaments extends out of the A band and into the less dense staining I bands. The lighter H bands in the center of the A bands are the regions where, when the muscle is relaxed, the thin filaments do not overlap the thick filaments. The Z lines allow for anchoring of the thin filaments. If a transverse section through the A band is examined under the electron microscope, each thick filament is seen to be surrounded by six thin filaments in a regular hexagonal pattern.
FIGURE 5–2
Skeletal muscle sarcomere. A) Electron micrograph of human gastrocnemius muscle (× 13,500). The sarcomere, named bands, and lines are shown. (Used with permission from GM Walker and GR Schrodt.) B) Arrangement of thin (actin) and thick (myosin) filaments and the Z line in a relaxed skeletal muscle. C) Arrangement of thin and thick filaments and the Z-line in a contracted skeletal muscle. Note that the Z-lines come together as the thick and thin filaments slide next to each other during contraction. The thick and think filaments do not change in size.
The form of myosin found in muscle is myosin-II, with two globular heads and a long tail. The heads of the myosin molecules form cross-bridges with actin. Myosin contains heavy chains and light chains, and its heads are made up of the light chains and the amino terminal portions of the heavy chains. These heads contain an actin-binding site and a catalytic site that hydrolyzes adenosine triphosphate (ATP). The myosin molecules are arranged symmetrically on either side of the center of the sarcomere, and it is this arrangement that creates the light areas in the pseudo-H zone. The M line is the site of the reversal of polarity of the myosin molecules in each of the thick filaments. At these points, there are slender cross-connections that hold the thick filaments in proper array. Each thick filament contains several hundred myosin molecules.
The thin filaments are polymers made up of two chains of actin that form a long double helix. Tropomyosin molecules are long filaments located in the groove between the two chains in the actin. Each thin filament contains 300–400 actin molecules and 40–60 tropomyosin molecules. Troponin molecules are small globular units located at intervals along the tropomyosin molecules. Each of the three troponin subunits has a unique function: Troponin T binds the troponin components to tropomyosin, troponin I inhibits the interaction of myosin with actin, and troponin C contains the binding sites for the Ca2+ that helps initiate contraction.
Some additional structural proteins that are important in skeletal muscle function include actinin, titin, and desmin. Actinin binds actin to the Z lines. Titin, the largest known protein (with a molecular mass near 3,000,000 Da), connects the Z lines to the M lines and provides scaffolding for the sarcomere. It contains two kinds of folded domains that provide muscle with its elasticity. At first when the muscle is stretched there is relatively little resistance as the domains unfold, but with further stretch there is a rapid increase in resistance that protects the structure of the sarcomere. Desmin adds structure to the Z lines in part by binding the Z lines to the plasma membrane. Some muscle disorders associated with these structural components are described in Clinical Box 5–1. It should be noted that although these proteins are important in muscle structure/function, by no means do they represent an exhaustive list.
The muscle fibrils are surrounded by structures made up of membranes that appear in electron micrographs as vesicles and tubules. These structures form the sarcotubular system, which is made up of a T system and a sarcoplasmic reticulum. The T system of transverse tubules, which is continuous with the sarcolemma of the muscle fiber, forms a grid perforated by the individual muscle fibrils (Figure 5–1). The space between the two layers of the T system is an extension of the extracellular space. The sarcoplasmic reticulum, which forms an irregular curtain around each of the fibrils, has enlarged terminal cisterns in close contact with the T system at the junctions between the A and I bands. At these points of contact, the arrangement of the central T system with a cistern of the sarcoplasmic reticulum on either side has led to the use of the term triads to describe the system. The T system, which is continuous with the sarcolemma, provides a path for the rapid transmission of the action potential from the cell membrane to all the fibrils in the muscle. The sarcoplasmic reticulum is an important store of Ca2+ and also participates in muscle metabolism.
CLINICAL BOX 5–1 Structural & Metabolic Disorders in Muscle Disease
The term muscular dystrophy is applied to diseases that cause progressive weakness of skeletal muscle. About 50 such diseases have been described, some of which include cardiac as well as skeletal muscle. They range from mild to severe and some are eventually fatal. They have multiple causes, but mutations in the genes for the various components of the dystrophin–glycoprotein complex are a prominent cause. The dystrophin gene is one of the largest in the body, and mutations can occur at many different sites in it. Duchenne muscular dystrophy is a serious form of dystrophy in which the dystrophin protein is absent from muscle. It is X-linked and usually fatal by the age of 30. In a milder form of the disease, Becker muscular dystrophy, dystrophin is present but altered or reduced in amount. Limb-girdle muscular dystrophies of various types are associated with mutations of the genes coding for the sarcoglycans or other components of the dystrophin–glycoprotein complex.
Due to its enormous size and structural role in the sarcomere, titin is a prominent target for mutations that give rise to muscle disease. Mutations that encode for shorter titin structure have been associated with dilated cardiomyopathy, while other mutations have been associated with hypertrophic cardiomyopathy. The skeletal muscle—associated tibialis muscular dystrophy is a genetic muscle disease of titin that is predicted to destabilize the folded state of the protein. Interestingly, many of the titin mutations identified thus far are in regions of titin that are expressed in all striated muscles, yet, not all muscles are affected in the same way. Such muscle type-specific phenotypes underscore the need to study titin’s multiple functions in different muscles, under both normal and pathologic conditions.
Desmin-related myopathies are a very rare heterogeneous group of muscle disorders that typically result in cellular aggregates of desmin. Common symptoms of these diseases are failing and wasting in the distal muscles of the lower limbs that can later be identified in other body areas. Studies in desmin knockout mice have revealed defects in skeletal, smooth, and cardiac muscle, notably in the diaphragm and heart.
Metabolic MyopathiesMutations in genes that code for enzymes involved in the metabolism of carbohydrates, fats, and proteins to CO2 and H2O in muscle and the production of ATP can cause metabolic myopathies (eg, McArdle syndrome). Metabolic myopathies all have in common exercise intolerance and the possibility of muscle breakdown due to accumulation of toxic metabolites.
THERAPEUTIC HIGHLIGHTSAlthough acute muscle pain and soreness can be treated with anti-inflammatory drugs and rest, the genetic dysfunctions described above are not as easily addressed. The overall goals are to slow muscle function/structure loss and, when possible relieve symptoms associated with the disease. Extensive monitoring, physical therapy, and appropriate drugs including corticosteroids can aid to slow disease progression. Assistive devices and surgery are not uncommon as the diseases progress.
The large dystrophin protein (molecular mass 427,000 Da) forms a rod that connects the thin actin filaments to the transmembrane protein β-dystroglycan in the sarcolemma by smaller proteins in the cytoplasm, syntrophins. β-dystroglycan is connected to merosin (merosin refers to laminins that contain the α2 subunit in their trimeric makeup) in the extracellular matrix by α-dystroglycan (Figure 5–3). The dystroglycans are in turn associated with a complex of four transmembrane glycoproteins: α-, β-, γ-, and δ-sarcoglycan. This dystrophin–glycoprotein complex adds strength to the muscle by providing a scaffolding for the fibrils and connecting them to the extracellular environment. Disruption of these important structural features can result in several different muscular dystrophies (see Clinical Box 5–1).
FIGURE 5–3
The dystrophin–glycoprotein complex. Dystrophin connects F-actin to the two members of the dystroglycan (DG) complex, α and β-dystroglycan, and these in turn connect to the merosin subunit of laminin 211 in the extracellular matrix. The sarcoglycan complex of four glycoproteins, α-, β-, γ-, and δ-sarcoglycan, sarcospan, and syntropins are all associated with the dystroglycan complex. There are muscle disorders associated with loss, abnormalities, or both of the sarcoglycans and merosin. (Used with permission of Justin Fallon and Kevin Campbell.)
ELECTRICAL PHENOMENA & IONIC FLUXES
The electrical events in skeletal muscle and the ionic fluxes that underlie them share distinct similarities to those in nerve, with quantitative differences in timing and magnitude. The resting membrane potential of skeletal muscle is about –90 mV. The action potential lasts 2–4 ms and is conducted along the muscle fiber at about 5 m/s. The absolute refractory period is 1–3 ms long, and the after-polarizations, with their related changes in threshold to electrical stimulation, are relatively prolonged. The initiation of impulses at the myoneural junction is discussed in the next chapter.
The distribution of ions across the muscle fiber membrane is similar to that across the nerve cell membrane. Approximate values for the various ions and their equilibrium potentials are shown in Table 5–1. As in nerves, depolarization is largely a manifestation of Na+ influx, and repolarization is largely a manifestation of K+ efflux.
Concentration (mmol/L) | |||
---|---|---|---|
Iona | Intracellular Fluid | Extracellular Fluid | Equilibrium Potential (mV) |
Na+ | 12 | 145 | +65 |
K+ | 155 | 4 | –95 |
H+ | 13 × 10–5 | 3.8 × 10–5 | –32 |
Cl– | 3.8 | 120 | –90 |
HCO3– | 8 | 27 | –32 |
A– | 155 | 0 | … |
Membrane potential = –90 mV |
CONTRACTILE RESPONSES
It is important to distinguish between the electrical and mechanical events in skeletal muscle. Although one response does not normally occur without the other, their physiologic bases and characteristics are different. Muscle fiber membrane depolarization normally starts at the motor endplate, the specialized structure under the motor nerve ending. The action potential is transmitted along the muscle fiber and initiates the contractile response.
A single action potential causes a brief contraction followed by relaxation. This response is called a muscle twitch. In Figure 5–4, the action potential and the twitch are plotted on the same time scale. The twitch starts about 2 ms after the start of depolarization of the membrane, before repolarization is complete. The duration of the twitch varies with the type of muscle being tested. “Fast” muscle fibers, primarily those concerned with fine, rapid, precise movement, have twitch durations as short as 7.5 ms. “Slow” muscle fibers, principally those involved in strong, gross, sustained movements, have twitch durations up to 100 ms.
FIGURE 5–4
The electrical and mechanical responses of a mammalian skeletal muscle fiber to a single maximal stimulus. The electrical response (mV potential change) and the mechanical response (T, tension in arbitrary units) are plotted on the same abscissa (time). The mechanical response is relatively long-lived compared to the electrical response that initiates contraction.
The process by which the contraction of muscle is brought about is a sliding of the thin filaments over the thick filaments. Note that this shortening is not due to changes in the actual lengths of the thick and thin filaments, rather, by their increased overlap within the muscle cell. The width of the A bands is constant, whereas the Z lines move closer together when the muscle contracts and farther apart when it relaxes (Figure 5–2).
The sliding during muscle contraction occurs when the myosin heads bind firmly to actin, bend at the junction of the head with the neck, and then detach. This “power stroke” depends on the simultaneous hydrolysis of ATP. Myosin-II molecules are dimers that have two heads, but only one attaches to actin at any given time. The probable sequence of events of the power stroke is outlined in Figure 5–5. In resting muscle, troponin I is bound to actin and tropomyosin and covers the sites where myosin heads interact with actin. Also at rest, the myosin head contains tightly bound adenosine phosphate (ADP). Following an action potential, cytosolic Ca2+ is increased and free Ca2+ binds to troponin C. This binding results in a weakening of the troponin I interaction with actin and exposes the actin binding site for myosin to allow for formation of myosin/actin cross-bridges. Upon formation of the cross-bridge, ADP is released, causing a conformational change in the myosin head that moves the thin filament relative to the thick filament, comprising the cross-bridge “power stroke.” ATP quickly binds to the free site on the myosin, which leads to a detachment of the myosin head from the thin filament. ATP is hydrolyzed and inorganic phosphate (Pi) released, causing a “re-cocking” of the myosin head and completing the cycle. As long as Ca2+ remains elevated and sufficient ATP is available, this cycle repeats. Many myosin heads cycle at or near the same time, and they cycle repeatedly, producing gross muscle contraction. Each power stroke shortens the sarcomere about 10 nm. Each thick filament has about 500 myosin heads, and each head cycles about five times per second during a rapid contraction.
FIGURE 5–5
Power stroke of myosin in skeletal muscle. A) At rest, myosin heads are bound to adenosine diphosphate and are said to be in a “cocked” position in relation to the thin filament, which does not have Ca2+ bound to the troponin—tropomyosin complex. B) Ca2+ bound to the troponin—tropomyosin complex induces a conformational change in the thin filament that allows for myosin heads to cross-bridge with thin filament actin. C) Myosin heads rotate, move the attached actin and shorten the muscle fiber, forming the power stroke. D) At the end of the power stroke, ATP binds to a now exposed site, and causes a detachment from the actin filament. E) ATP is hydrolyzed into ADP and inorganic phosphate (Pi) and this chemical energy is used to “re-cock” the myosin head. (Data from Huxley AF, Simmons RM: Proposed mechanism of force generation in striated muscle. Nature Oct 22;233(5321):533–538, 1971 and Squire JM: Molecular mechanisms in muscular contraction. Trends Neurosci 6:409–413, 1093.)
The process by which depolarization of the muscle fiber initiates contraction is called excitation–contraction coupling. The action potential is transmitted to all the fibrils in the fiber via the T system (Figure 5–6). It triggers the release of Ca2+ from the terminal cisterns, the lateral sacs of the sarcoplasmic reticulum next to the T system. Depolarization of the T tubule membrane activates the sarcoplasmic reticulum via dihydropyridine receptors (DHPR), named for the drug dihydropyridine, which blocks them (Figure 5–7). DHPR are voltage-gated Ca2+ channels in the T tubule membrane. In cardiac muscle, influx of Ca2+ via these channels triggers the release of Ca2+ stored in the sarcoplasmic reticulum (calcium-induced calcium release) by activating the ryanodine receptor (RyR). The RyR is named after the plant alkaloid ryanodine that was used in its discovery. The RyR is a ligand-gated Ca2+ channel with Ca2+ as its natural ligand. In skeletal muscle, Ca2+ entry from the extracellular fluid (ECF) by this route is not required for Ca2+ release. Instead, the DHPR that serves as the voltage sensor unlocks release of Ca2+ from the nearby sarcoplasmic reticulum via physical interaction with the RyR. The released Ca2+ is quickly amplified through calcium-induced calcium release. Ca2+ concentration is reduced in the muscle cell by the sarcoplasmic or endoplasmic reticulum Ca2+ ATPase (SERCA). The SERCA pump uses energy from ATP hydrolysis to remove Ca2+ from the cytosol back into the terminal cisterns, where it is stored until released by the next action potential. Once the Ca2+ concentration outside the sarcoplasmic reticulum has been lowered sufficiently, chemical interaction between myosin and actin ceases and the muscle relaxes. Note that ATP provides the energy for both contraction (at the myosin head) and relaxation (via SERCA). If transport of Ca2+ into the sarcoplasmic reticulum is inhibited, relaxation does not occur even though there are no more action potentials; the resulting sustained contraction is called a contracture. Alterations in the excitable response in muscle underscore many different pathologies (Clinical Box 5–2).
FIGURE 5–7
Relation of the T tubule to the sarcoplasmic reticulum in Ca2+ transport. In skeletal muscle, the voltage-gated dihydropyridine receptor in the T tubule triggers Ca2+ release from the sarcoplasmic reticulum (SR) via the ryanodine receptor (RyR). Upon sensing a voltage change, there is a physical interaction between the sarcolemmal-bound DHPR and the SR-bound RyR. This interaction gates the RyR and allows for Ca2+ release from the SR.
Muscular contraction involves shortening of the contractile elements, but because muscles have elastic and viscous elements in series with the contractile mechanism, it is possible for contraction to occur without an appreciable decrease in the length of the whole muscle (Figure 5–8). Such a contraction is called isometric (“same measure” or length). Contraction against a constant load with a decrease in muscle length is isotonic (“same tension”). Note that because work is the product of force times distance, isotonic contractions do work, whereas isometric contractions do not. In other situations, muscle can do negative work while lengthening against a constant weight.
FIGURE 5–8
Isotonic and isometric contractions. A) Muscle preparation arranged for recording isotonic contractions. B) Preparation arranged for recording isometric contractions. In A, the muscle is fastened to a writing lever that swings on a pivot. In B, it is attached to an electronic transducer that measures the force generated without permitting the muscle to shorten.
The electrical response of a muscle fiber to repeated stimulation is like that of nerve. The fiber is electrically refractory only during the rising phase and part of the falling phase of the spike potential. At this time, the contraction initiated by the first stimulus is just beginning. However, because the contractile mechanism does not have a refractory period, repeated stimulation before relaxation has occurred produces additional activation of the contractile elements and a response that is added to the contraction already present. This phenomenon is known as summation of contractions. The tension developed during summation is considerably greater than that during the single muscle twitch. With rapidly repeated stimulation, activation of the contractile mechanism occurs repeatedly before any relaxation has occurred, and the individual responses fuse into one continuous contraction. Such a response is called a tetanus (tetanic contraction). It is a complete tetanus when no relaxation occurs between stimuli and an incomplete tetanus
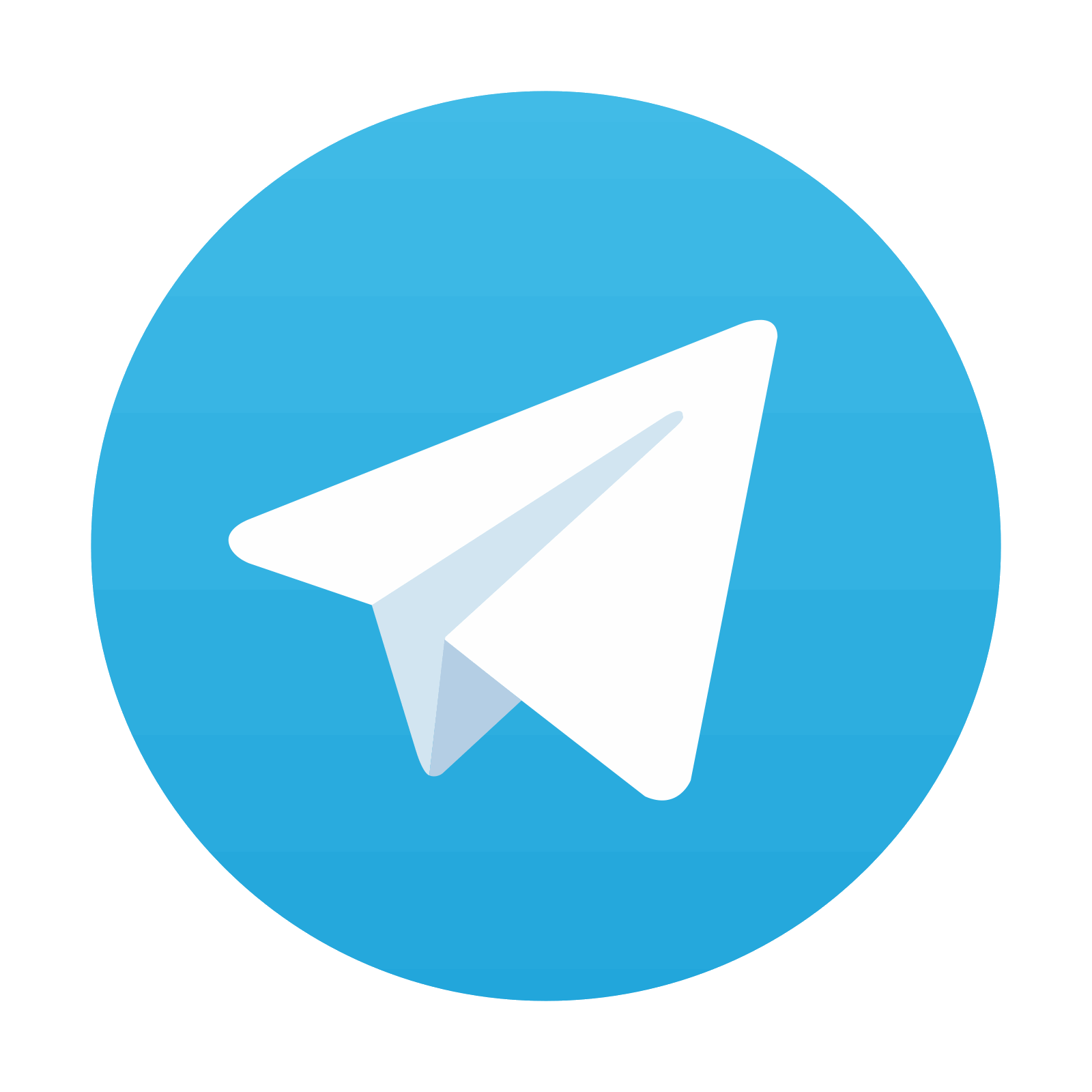
Stay updated, free articles. Join our Telegram channel
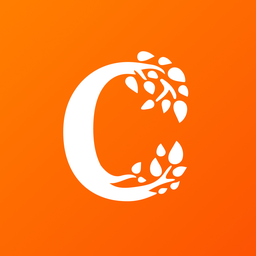
Full access? Get Clinical Tree
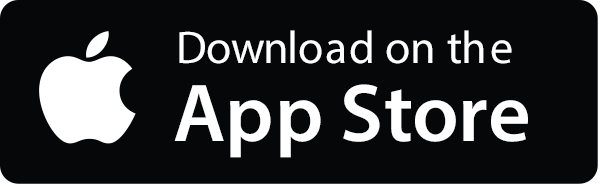
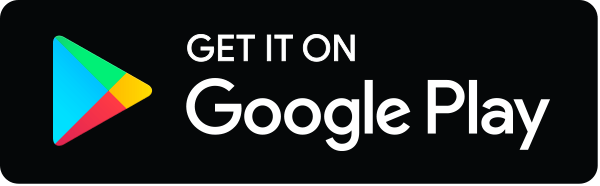
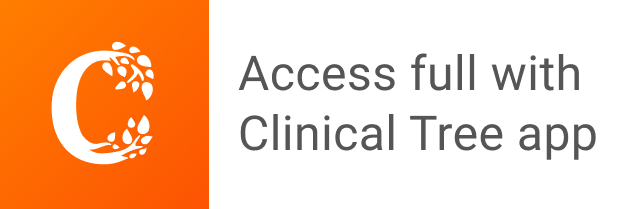