Fig. 19.1
Nanomaterials employed for theranostic applications
19.2 Synthesis of Nanoparticulate Raw Materials
19.2.1 Nobel Metal Nanoparticles
19.2.1.1 Gold
Gold and its compounds have long been used as medicinal agents, and their use can be tracked to ancient Egypt and China [1]. The Egyptians added gold to certain foods for spiritual and bodily purification 5,000 years ago. In 2500 BC, red colloidal gold was included in Chinese remedies for longer life. During the last century, gold has also been used to treat arthritis. Recently, gold nanoparticles (GNPs) have become an important biomedical material for cancer research due to their inert properties and non-toxic nature, ease of synthesis and functionalization, tunable size via synthetic parameters, and the surface plasma resonance (SPR) effect [2–6].
In general, well-dispersed GNPs can be prepared by reducing gold salts in the presence of appropriate reductants and stabilizing agents, a process that has been applied for over 50 years. The size and shape of the GNPs can be manipulated by varying temperature, concentration of the precursor, the type and concentration of the reductant, and the type and concentration of the stabilizing agents [5]. Well-dispersed gold colloids have been applied in bio-sensing, bio-labeling, targeted imaging, and photoacoustic imaging [7]. In order to achieve the targeting, sensing, and delivering functions, delivery systems based on GNPs-bearing functional moieties with thiol linkers have been developed. In the most common procedure for preparing GNPs, AuCl4 − salts are reduced with NaBH4 in the presence of the desired thiol-based capping agents. The size of the particles can be tuned from 1.5 to 6 nm by adjusting the stoichiometry of the thiol–gold reaction [8]. On the other hand, for some biomedical applications, such as photoacoustic imaging, gold nanorods, are required [9]. Gold nanorods can be synthesized by using the seed-mediated growth method. Jana et al. prepared colloidal gold nanorods by firstly forming citrate-capped gold seeds, which were subsequently added to a growth solution obtained by using ascorbic acid to reduce HAuCl4 in the presence of the surfactant cetyltrimethylammonium bromide (CTAB) and silver ions [10]. This method was modified by Nikoobakht et al. [11] in which the seed solution was obtained by using NaBH4 to reduce HAuCl4 in the presence of CTAB in an ice bath. This seed solution was then added to the Au growth solution obtained by using ascorbic acid to reduce HAuCl4 in the presence of CTAB and treated with AgNO3 prior to the mixing to facilitate the nanorod growth and to tune the aspect ratio.
19.2.1.2 Silver
Silver has been used as an antimicrobial agent for thousands of years. Recently, silver nanoparticles have received considerable attention because of their SPR phenomenon, surface enhanced Raman scattering (SERS) effect, and catalytic property, in addition to antimicrobial activity [12, 13]. These properties of silver nanoparticles vary with their shape, size and formulation [14] which can be manipulated by a variety of synthetic methodologies using photo-, thermo-, sono-, wet-, bio- and electro-chemical principles [12]. For example, electrochemistry has been employed to prepare Ag nanowires, nanorods, nanocubes, and nanoplates by tuning parameters such as current density and Ag salt concentration. In order to further manipulate the nucleation and growth kinetics during the synthetic process, ultrasonication was employed to enhance mass transport and organic capping agents were used to selectively inhibit crystal growth in specific crystallographic planes. Organic capping reagents, such as the non-toxic poly(vinyl pyrrolidone) (PVP), are widely used in the shape-selective synthesis of NPs.
19.2.2 Quantum Dots: CdSe and CdTe
In general, QDs are defined as nanometer-sized crystals that demonstrate a quantum confinement effect when the nanoparticle radius is smaller than the Bohr radius of the electron, hole, and exciton. Typically, QD sizes range between 2 and 10 nm [15]. Recently, QDs have been intensively investigated because their photoluminescence properties are size-dependent and stable compared with those of organic fluorescent molecules, which are advantageous for bio-sensing, bio-labeling, and imaging [16]. However, most QDs without further surface modification are toxic, which limits their application for medical use (vide infra).
CdSe and CdTe are the most common QDs studied because their quantum confinement region covers the entire visible light spectrum range. The main cause of the toxicity of Cd-based QDs is the ability of released Cd2+ ions to bind to thiol groups in mitochondria, followed by considerable stress and damage that causes cell death. Hence, a variety of surface coatings have been developed to reduce the release of Cd2+ ions. One of the most common coating layers used is the amorphous SiO2 shell, which can be prepared by using a silica precursor, such as tetraethylorthosilicate (TEOS). The silica shell can further conjugate with other molecules or polymers to construct a hydrophilic, well-dispersed, and functionalized colloid system [17].
CdSe QDs can be prepared by the pyrolysis of organometallic precursors of Cd (e.g., dimethyl cadmium, CdMe2) and Se in trioctylphosphine (TOP) at 230–300 °C. Size-selective precipitation is employed to obtain mono-sized nanoparticles. This method was later modified because of the volatile, pyrophoric, and toxic nature of CdMe2. Alternative Cd precursors such as cadmium oxide and cadmium acetate have been used [18]. The QDs obtained through the thermal decomposition route are usually hydrophobic because the precursors are decomposed and reacted with capping agents in an oil-phase state. However, in most cases, hydrophilic, colloidal QDs dispersed in aqueous solution are preferred for intravascular, extravascular, and intracellular delivery. Therefore, many methods have been developed to synthesize hydrophilic QDs [19, 20]. In one method, the hydrophobic ligands are replaced with bifunctional molecules containing a soft acidic group (e.g., thioglycolic acid, TGA) and hydrophilic groups (e.g., –COOH or –NH2) to improve the colloid stability [21]. On the other hand, TGA has been used as a hydrophilic capping agent to synthesize CdTe QDs directly. In this process, first, aluminum telluride is mixed with a dilute sulfuric acid solution under N2 atmosphere to prepare H2Te. The resulting H2Te gas and an aqueous solution of cadmium perchlorate hexahydrate are then reacted in the presence of TGA at 100 °C and pH 11.5 to produce CdTe QDs.
19.2.3 Magnetic Nanoparticles: Fe3O4
Superparamagnetic iron oxide nanoparticles (SPIONs) have been used for a number of biomedical applications such as separation of biomoleculars, biosensing, targeted drug delivery, hyperthermia, magnetic-triggered release, and as magnetic resonance imaging (MRI) contrast agents. Several synthetic methods have been developed to prepare SPIONs employing microemulsions, sol–gel syntheses, sonochemical reactions, hydrothermal reactions, hydrolysis, and thermolysis of precursors [22–24].
The coprecipitation method is the simplest way to prepare Fe3O4 (magnetite) nanoparticles in which an aqueous mixture of Fe(II) and Fe(III) chloride at a 1:2 molar ratio is added a base in a non-oxidizing oxygen environment at pH 9–14. For example, highly monodisperse SPIONs can be synthesized by utilizing water-in-oil microemulsions in which the aqueous core of aerosol-OT (AOT)/n-hexane reverse micelles is formed to dissolve hydrophilic precursors in a deoxygenated sodium hydroxide solution. Chemical precipitation occurs in the reverse micelles (i.e., nanoreactors), which leads to the formation of SPIONs with diameter less than 15 nm.
On the other hand, iron organic precursors, such as Fe(CO)5 and Fe(acac)3, have been used to prepare mono-sized and well-dispersed nanoparticles in octyl ether and oleic acid at high temperature. In another process, Fe(CO)5 is decomposed at 100 °C in the presence of oleic acid and then aged at 300 °C to yield high crystallinity monodisperse maghemite (γ-Fe2O3) in oil phase. These high temperature thermolysis reactions are normally carried out under sonication to generate high temperature hot spot, i.e., ultrasound-induced cavitation. For example, an aqueous solution of Fe(CO)5 mixed with sodium dodecyl sulfate under sonication yielded amorphous Fe3O4 nanoparticles.
19.2.4 Bioactive Nanoparticles: Hydroxyapatite
Nanomaterials can also be found in animals. For example, bone is composed of calcium phosphate (with nanosized crystals) and collagen (with nanosized structure) by which the high strength/weight ratio can be achieved to mechanically protect and support the body. Hydroxyapatite (HAp) is one type of calcium phosphate and the major inorganic component of bone mineral [25]. The calcium-deficient apatitic structure (Ca-deficient hydroxyapatite, CDHA) is also an essential component of natural bone mineral. CDHA is compositionally similar to tricalcium phosphates (Ca/P = 1.5) and structurally similar to stoichiometric hydroxyapatite (Ca/P = 1.67) [26]. Therefore, HA and CDHA are considered to be candidate materials for orthopedic applications with respect to both mechanical reinforcement and biological activity [27]. In the human body, the nanosized HAp crystals (generally 30–50 nm in length, 15–30 nm in width, and 2–10 nm in thickness) play an important role in the strength (resistance to deformation) and toughness (resistance to flaw propagation) of the bone. In addition, nanosized HAp crystals act as Ca and P reservoirs for many metabolic processes in the human body.
Many synthetic routes have been developed to prepare HAp nanoparticles including sol–gel, co-precipitation and hydrothermal methods [28, 29]. Among these methods, co-precipitation is the most common wet-chemical method to prepare HAp nanoparticles [30]. In this method, HAp nanoparticles are obtained by mixing aqueous solutions of a number of precursor compounds containing Ca2+ [e.g., CaCl2, Ca(NO3)2, Ca(OH)2, CaCO3, CaSO4·2H2O, and Ca(CH3COO)2] and PO4 3− [e.g., H3PO4, NH4H2PO4, (NH4)2HPO4, Na3PO4, and K3PO4] ions at a Ca/P ratio of 1.67 and pH values of 7–14. The pH of the reaction solution is one of the most important parameters affecting the morphology and crystallinity of HA nanoparticles by changing the rate and extent of the precursor hydrolysis reaction which can be adjusted by adding ammonia gas, NH4OH, or NaOH. In addition to pH, other parameters such as the concentrations of the precursor salts, mixing sequence and rate, reaction temperature, and aging time all affect the morphology and crystallinity of HA nanoparticles. To further manipulate the morphology of HA nanoparticles, the synthetic reaction could be restricted in nanosized reaction vessels, such as reverse micelles, microemulsions, and vesicles. For example, poly(allylamine hydrochloride)/PO43- complex was employed as spatial reaction vessels and a source of phosphate anions to capture calcium cations to synthesize HAp.
19.3 Polymer-Based Nanoparticles
19.3.1 The Physiological Itinerary of Nanoparticles
The use of nanoparticles efficiently to deliver to targeted tissues for diagnosis or therapy requires to fulfill three major concerns that are associated with physiological conditions. The first is to prolong the circulation half-life of the nanoparticles, to which the main barriers are believed to be the interactions with blood components, filtration by the kidney, recognition by the reticuloendothelial system (RES) in the liver, spleen, and lung [31], as well as clearance by the monophagocytic system (MPs) [32]. It is known that both physical properties (e.g., particle size, morphology, shape, and hydrophobicity) and chemical properties (e.g., surface chemistries such as charges and modifications) of the nanoparticles can affect directly their absorption of plasma proteins, recognition by the RES and elimination from the blood [33–35]. For example, microparticles with diameters 1–10 μm could be directly captured by liver’s Kupffer cells, whereas nanoparticles below 100 nm were recognized for extravasation from blood into targeted tissues [36, 37]. For the effect of shape on circulation, cylindrical particles showed negligible phagocytosis and prolonged circulation as compared with spherical particles under the same volume [38]. However, by surface-chemical modification, gold nanorods in combination with various polymer grafting levels exhibited different biodistribution results in liver, lung, and spleen [39]. Zhang et al. evaluated the effect of particle size (45–90 nm) on the in vivo pharmacokinetics and biodistribution [40]. Their results demonstrated that 90 nm particles were taken up more rapidly than 45 and 60 nm particles by RES cells.
The second concern is to deliver nanoparticles from blood through permeation to targeted tumor tissues and accumulate there. The accumulation of nanoparticles in tumors can be achieved by the microvascular hyperpermeability due to vast angiogenesis and localized leaky vasculature, called the “enhanced permeability and retention (EPR) effect” [41, 42]. The pore cutoff size in most solid tumors studied is in general in the range 380–780 nm, beyond which the nanoparticles cannot be accumulated in the tumor tissue [43]. Perrault et al. reported the effect of nanoparticle size from 10 to 100 nm on the passive targeting onto tumors and found that PEG-modified GNPs having 40–100 nm diameter would provide excellent tumor accumulation [44]. Normally, smaller nanoparticles had relatively higher permeation rate from vasculature to tumors and vice versa for their clearance. If the goal is to deliver a nanoparticulate contrast agent to tumors, Perrault et al. suggested that moderate sized particles having a final diameter of 60–100 nm after mPEG modification would be preferred. However, if nanoparticles were to be used as drug carriers for cancer therapy for which longer localization within the tumor mass is important, it is better to use an active tumor targeting process. This can be achieved by chemically modifying ligands on the surfaces of nanoparticles to allow selective binding to receptors that are specifically expressed on the membranes of cancer cells.
The third concern is the uptake of nanoparticles by the cancer cells following accumulation, if nanoparticles are designed for intracellular delivery. In general, the internalization of nanoparticles into cells could be done by either pinocytosis or receptor-mediated endocytosis. Unlike receptor-mediated endocytosis which uptakes nanoparticles via the interaction of ligands with specific receptors on the cellular membrane, pinocytosis is a low efficiency and non-specific transport pathway. In addition to cell type, nanoparticle surface properties such as ligands modification, particle size and charge all influence the rate and accumulated efficiency of cellular uptake. Many studies of intracellular accumulation suggest that GNPs with diameters of approximately 40–60 nm exert the fastest wrapping time and moderate exocytosis and therefore accumulate the most in cancer cells [45–49]. It is known that positively charged nanoparticles activate a compensatory endocytosis pathway that also enhances the accumulation of nanoparticles in cells [50]. These results all suggest that the physical and chemical properties of nanoparticles strongly influence the circulation half-live, biodistribution, tumor accumulation, and cellular internalization.
19.3.2 Polymers in Nanoparticles Modification
The most direct method to alter the physical and chemical properties of nanoparticles to enhance the accumulation in targeted cells is to modify them by hydrophilic polymers (i.e., passive targeting) and decorated with specific targeting ligands (i.e., active targeting). GNPs could be used as a model system for describing the biodistribution of particles, vasculature permeation, and cell internalization for polymer modification. De Jong et al. [51] studied the particle size dependence on the distribution in organs of intravenously injected GNPs. Their results revealed that naked GNPs of sizes 10, 50, 100, and 250 nm were all present in the liver and spleen. However, the smallest (10 nm) nanoparticles were also widely distributed in various other organs, including kidney, testis, thymus, heart, lung, and brain which might create problems if specific targeting to tumors is desired. Lipka et al. [52] showed that modifying 5 nm GNPs with PEG affected their pharmacokinetics, especially prolonging their circulation time and reducing their uptake by the liver, spleen, and other organs. Similar to GNPS, single-walled carbon nanotubes (SWNTs) coated with PEG showed relatively long circulation time and low RES uptake, as compared with un-modified SWNTs [53]. Also, dextran-coated iron oxide nanoparticles with PEG and chimeric L6 antibody modification exhibited long circulation time and ability to exit from blood vessels and access the cancer cells [54].
Besides directly changing the biodistribution and organ accumulation, nanoparticles modified by biocompatible hydrophilic polymers can also decrease their cellular cytotoxicity which is also of prime concern if these materials are to be used clinically. For example, CdSe QDs have been used for a number of animal in vivo imaging studies before extensive cytotoxicity studies, however, bare CdSe QDs undergo surface oxidation when incubated with cells and induce the release of Cd(II) ions, which is known to damage mitochondrial and lead to cell death [55]. One method to prevent Cd(II) ions releasing from CdSe QDs is to coat an inner ZnS shell onto their surface together with a polymer (e.g., PEG) outer layer. Another example to reduce cytotoxicity is to use the hydrophilic pullulan (Pn) polymer to modify the surface of SPIONs [56]. It has been reported that naked SPIONs may promote cell apoptosis and lead to low cell viability. With Pn-coating, the modified SPIONs exhibit significantly lower cytotoxicity than naked particles.
The biocompatible hydrophilic polymers used for nanoparticle surface modification include PEG, dextran, and chitosan. PEG is the most important and widely used hydrophilic and water soluble polymer so far. It has been approved by FDA for parenteral applications because of its better understood biocompatibility and safety properties. The molecular weights of PEG for safe parenteral administration range from 1 to 20 kDa. Several reports have showed the use of PEG on inorganic nanoparticles to increase the biocompatibility and blood circulation time [57–59]. In addition to PEG, dextran is a hydrophilic polysaccharide also often used for polymer coating with a well-known history of safe applications. For example, dextran has been used to coat SPIONs (e.g., Ferridex®, a FDA approved MRI contrast agent) to enhance particle stability and to prolong blood circulation time [60]. On the other hand, chitosan is a non-toxic, biocompatible, biodegradable, and muco-adhesive polymer. The use of chitosan-modified magnetic nanoparticles has generated some research interests [61, 62]. For example, chitosan encapsulating nanoparticles comprising both CdSe-ZnS QDs and GdDTPA have been reported as potential dual-modality fluorescent biomarkers and MRI contrast agents [63]. These kinds of multifunctional nano-medicines are considered as promising candidates for future biomedical applications.
19.3.3 Surface Engineering of Inorganic Nanoparticles by Polymers
For surface modification of nanoparticles, direct linkage of polymers by covalent coupling and coating polymers by non-covalent immobilization are two major used strategies. Non-covalent immobilization is also known as physical adsorption which results from molecular interactions by Van der Waals and/or electrostatic forces, molecular affinity, and polymer crosslinking after adsorption. Polycyclic aromatic compounds could be immobilized onto the surface of carbon nanotubes through Van der Waals forces [64]. An electrostatic interaction has been used to link positively charged enzymes and negatively charged CdTe QDs [65]. Molecules such as glutathione with disulfide bonds and PEG derivatives with thiol functional groups have been conjugated directly to gold surfaces by their strong affinities to gold atoms [44, 66]. Such interactions between thiols and metal atoms are also called semi-covalent interaction.
For covalent binding of polymer on inert solid nanoparticles, the surface must firstly be chemically treated to provide reactive function groups (e.g., hydroxyl (–OH), amine (–NH2), carboxyl (–COOH), etc.) for the subsequent immobilization steps. These surface functional groups can be generated by oxidation, hydrolysis, and radiation. After the surface pretreatment, polymer immobilization on nanoparticles by covalent coupling can be performed through peptide, ester or other bond formation. Scheme 19.1 illustrates some examples of covalent surface modification. For example, the hydroxyl end-capped group can react with aminosilane, tresylchloride, cyanogen bromide or p-nitrophenyl chloroformate to form an active intermediate. Thionyl chloride, phosgene, butanediol, or bis(2,5-dioxopyrrolidin-1-yl) carbonate can be used to prepare active intermediates with an end-capped amine group. For the carboxyl group, the active intermediate can be synthesized with thionyl chloride or N-hydroxysuccinimide. The immobilization of polymer on nanoparticles can then be performed by appropriate specific reaction with the active intermediate.
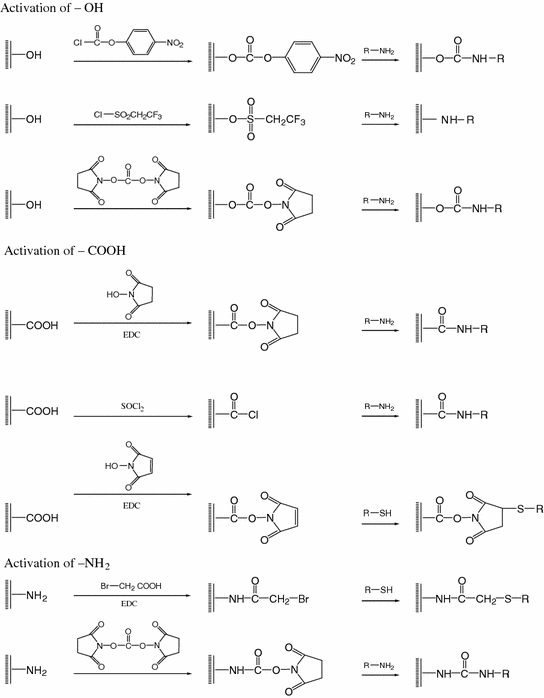
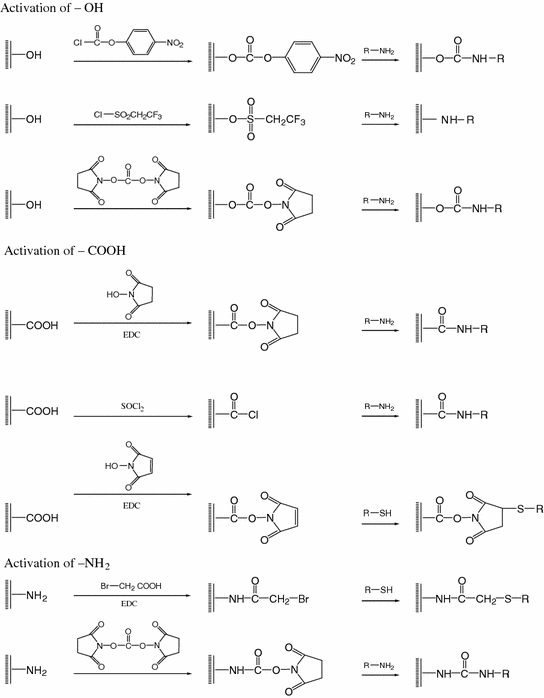
Scheme 19.1
Covalent surface modification of solid nanoparticles
19.4 Surface-Modified Nano-Biosensors
19.4.1 Surface-Modified Gold Nanoparticle-Based Colorimetric Biosensing
Over the past several decades, the modulation of Au nanomaterials through dispersion-aggregation conversion process has been developed as a colorimetric sensing platform for rapid and cost-effective bioanalysis and environmental monitoring. Spherical Au nanoparticles exerted localized SPR absorption [67]. For example, 13-nm-sized Au nanoparticles have strong and single SPR peak at 520 nm with high extinction coefficient (2.7 × 108 M−1 cm−1). The dispersed colloidal solution of Au nanoparticles has a red color with over 100 time greater intensity than most organic dyes. In the presence of specific analyte molecules of interest, the Au nanoparticles could aggregate and the red color converts to purple due to shifting of SPR band maximum to longer wavelength [67, 68].
The degree of dispersion and/or aggregation process of the Au nanomaterials can be successfully manipulated by using similar or different sizes and/or shapes of Au nanoparticles in the form of nanospheres, nanorods, and nanosphere/nanorod mixtures which self-assemble in the presence of analyte molecules (Fig. 19.2) [69–78]. For this reason, specific analyte molecules could be determined by different colors resulted from this manipulation. In 1997, Mirkin and co-workers firstly reported the detection of specific polynucleotides through the hybridization of their Au nanoparticle derivatives with known-sequence DNA-modified Au nanoparticles [71]. The Thiol (–SH) functional groups on the polynucleotides have good affinity to immobilize on the Au nanoparticle surfaces by the formation of Au–S bonds. A significant color change from red to blue-purple was found when the polynucleotides hybridize with the known-sequence DNA molecules. The color change was reversible if the surrounding temperature was increased to the melting temperature of the polynucleotide-DNA hybrid. This method is more sensitive compared with the traditional one based on the DNA absorption intensity changes at 260 nm.
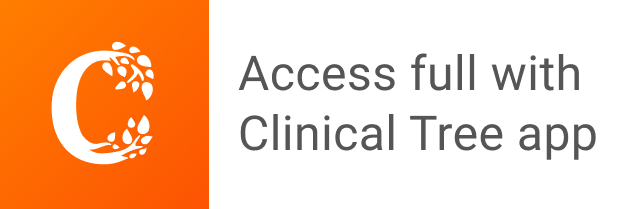