Fig. 1.1
An outline of this book, which is organized into 5 parts
Part I of this book, which includes Chaps. 2–7, is focused on cell and tissue engineering. Regenerative medicine holds tremendous potential in the treatment of a wide variety of human diseases; for many of those, it may be the only solution [3]. In Chap. 2, Dr. Ray and co-workers discussed about these “therapeutic wonders” (i.e., stem cells) and reviewed the ongoing investigations on stem cell biology, as well as their clinical applicability which can bring revolutions to future clinical care. Engineering of stem cells, non-invasive imaging of stem cells, as well as clinical applications of stems cells in a number of human diseases were all covered in this chapter. In Chap. 3, Dr. Cooper and co-workers focused on the treatment of cancer with engineered T cells. Since tumor microenvironment is often immune suppressive, which protects cancer cells from recognition and elimination by effector cells, engineered T cells can be used for effective cancer cell killing. This chapter provided a comprehensive summary of adoptive cell therapy with genetically modified T cells to redirect specificity to tumor cell antigens using a number of approaches, to improve T-cell effector function, as well as to control these T cells with suicide genes to ensure safety.
Much recent research effort has been devoted to the development of various scaffold systems that can carry cells, using a broad array of biomaterials. In Chap. 4, Dr. Wang and his co-worker gave a thorough review on engineering biomaterials for the delivery of anchorage-dependent and non-anchorage-dependent therapeutic cells. Some cells (e.g., muscle cells and neurons) are dependent on anchorage to the extracellular matrix. Therefore, they require extensive cell adhesion to a substrate to survive and elicit biological function upon transplantation. On the other hand, non-anchorage-dependent cells (e.g., chondrocytes and hepatocytes) do not have such requirement, which often exhibit a rounded morphology in their native environment. Clearly, different cell delivery structures are needed for these cells to achieve maximal therapeutic effect, which were illustrated in detail in this chapter.
The next three chapters in Part I of this book are focused on tissue repair. Peripheral nerve injuries can lead to variable levels of functional loss, depending on the extent of injury. In Chap. 5, Dr. Orbay and I had a detailed survey into the components of tissue-engineered nerve grafts, as well as reviewed the relevant clinical studies. A tissue-engineered nerve is typically composed of a biodegradable scaffold, a neurogenic cell line, and growth factors. Therefore, how to improve the cell–scaffold and scaffold–tissue interactions is a key challenge for peripheral nerve repair. Perhaps even more important than nerve repair, the establishment of blood vessel networks is a matter of life and death for tissues and organisms. In Chap. 6, Dr. Murphy and co-workers discussed about the structure of blood vessels and key signaling molecules, which play significant role in vasculogenesis, angiogenesis, and maturation of nascent blood vessels. A description of promising approaches specific to tissue-engineered blood vessels, various tissue engineering approaches to identify the appropriate sources of endothelial cells, a brief introduction to some clinical results, as well as the regulatory challenges were all included in this chapter. Cartilage tissue is prone to significant dysfunction after damage, which also lacks the innate ability to effectively repair itself. Chapter 7, written by Dr. Zhang and his co-worker, was focused on engineering gene-activated matrices for the repair of articular cartilage defect. Although growth factors were shown to promote cartilage repair, the structure of cartilage tissue can hinder direct addition of growth factors into the traditional system, due to the difficulty in controlling the amount of growth factors added and the short biological half-lives. Therefore, gene-activated matrix systems have been developed for the repair of articular cartilage damage, which can secret large quantity of growth factors continuously and serve as a promising therapeutic approach for repairing cartilage damage.
The ten following chapters in Part II of this book cover a broad array of areas related to genetic and protein engineering. Many luciferases have been isolated and investigated for broad biomedical applications, ranging from cell-based studies to in vivo applications in small animal models [4, 5]. In Chap. 8, Dr. Walls and Dr. Loening provided an excellent and comprehensive review of engineered luciferases. They discussed in detail about how protein engineering can be used to improve their stability, light output, emission wavelength, chemical sensitivity, and substrate specificity among others for better in vitro/in vivo performances and broader biomedical applications. After significant improvement and optimization of the naturally occurring luciferases (e.g., firefly luciferase and Renilla luciferase, as discussed in Chap. 8) and other enzymes (e.g., herpes simplex virus type-1 thymidine kinase), they can be employed for a wide variety of biomedical applications, two of which are detailed in the two following chapters.
In Chap. 9, Dr. Massoud and Dr. Paulmurugan focused on the use of engineered split reporter systems for in vivo molecular imaging of protein–protein interactions, which can pave the way for functional proteomics in living animals and provide a tool for whole-body evaluation of new pharmaceuticals that can modulate protein–protein interactions. The main strategies currently available for imaging protein–protein interactions in living subjects using molecularly engineered and rationally designed split reporter gene, as well as the broad (potential) uses of these strategies, were illustrated in exquisite detail. A few of the major strategies adopted for split reporter gene-based imaging include intein-mediated reconstitution and protein fragment complementation (which can be achieved via a variety of different mechanisms), which can enable the interrogation of many protein–protein interactions through in vitro assays and/or non-invasive imaging. Besides split reporter gene techniques, bioluminescence resonance energy transfer (BRET)-based sensors are rapidly expanding, which can also be adopted for investigation of protein–protein interactions, protein dimerization, signal transduction, etc. [6, 7]. In Chap. 10, Dr. De and co-workers presented an in-depth overview on the engineering requirements of BRET components such as donor, acceptor, substrate chemistry, and instrumentation. Both genetically engineered and synthetic BRET systems were described, which have witnessed significant advancement over the last decade and can be used in a variety of systems including both in vitro assays and in vivo applications.
Besides luciferases and other enzymes, a variety of other engineered proteins can be used for biomedical applications, which are the topics of the subsequent 4 chapters. Antibodies are a major constituent of the human immune system and have become an indispensable class of therapeutics in cancer and many other diseases [8, 9]. In Chap. 11, Dr. Fischer summarized the topic of antibody engineering in translational medicine, which offered an overview of current strategies to tailor antibodies for biomedical applications. After a brief introduction, this chapter covers many related topics which include approaches to reduce immunogenicity of therapeutic antibodies, optimize pharmacokinetics, and enhance therapeutic efficacy (e.g., with Fc engineering to improve effector function, generation of antibody–drug conjugates, and radioimmunoconjugates). In addition, engineered antibody fragments and bispecific antibodies were also discussed, which can be advantageous than intact antibodies for many applications due to the much shorter circulation half-life and other desirable properties (e.g., the capability to bind to two different antigens simultaneously). Together with the conventional antibodies, many of which have been approved for clinical use over the last two decades, these novel engineered antibody-based imaging agents and therapeutics will have a major impact on future diagnosis and treatment of many diseases, including but not limited to cancer.
Affibodies are an emerging class of small (7 kDa) protein scaffold-based affinity ligands, which have attracted much attention recently and are the focus of Chap. 12, written by Dr. Cheng and co-workers. Because of the small size (58 amino acid residues), affibodies can be readily produced via both peptide synthesis and recombinant expression in Escherichia coli. General structures and engineering strategies used to optimize affibody molecules for imaging and therapy applications are described in this chapter. As evidenced by the constantly emerging literature reports on this class of exciting proteins, affibodies have been extensively investigated for imaging applications with different techniques (e.g., upon labeling with various radionuclides for positron emission tomography or single-photon emission computed tomography, fluorescent dyes or quantum dots for optical imaging, and magnetic nanoparticles for magnetic resonance imaging), and most of the current research effort is focused on cancer research. In addition, affibodies have been explored for therapeutic applications as well (e.g., upon labeling with 177Lu or 90Y, or used as targeting ligands to redirect cytotoxic T lymphocytes and natural killer cells). With recent clinical studies indicating safety and efficacy of affibody-based imaging agents, affibodies hold promising potential in future clinical patient management.
In Chap. 13, Dr. Hackel provided a comprehensive summary of various protein scaffolds that can be used for molecular imaging and therapy applications. Since effective targeting of imaging/therapeutic agents requires specific binding with high-affinity, facile and robust conjugation of effectors (e.g., drugs, genes, radioisotopes, and therapeutic proteins) without compromising the binding/biological activity, and efficient in vivo delivery, protein scaffolds can serve as promising platforms for these applications. Aside from affibodies that were covered in Chap. 12, other validated scaffolds include the fibronectin domain, knottin, designed ankyrin repeat protein, anticalin, etc. With clinical trials in therapy or imaging already ongoing with many of these scaffolds, the future is bright for newly developed agents that are based on these protein scaffolds.
In Chap. 14, Dr. Cochran and Miss Liu focused on the topic of engineering multivalent and multispecific protein therapeutics. Since many proteins utilize the principles of multivalency and multispecificity to ensure optimal biological function in nature [10], their mechanisms of action have inspired the development of next-generation protein therapeutics with improved efficacy and safety profiles. The main advantages conferred by multivalency and multispecificity include increased target-binding affinity through avidity effects and selectivity for a diseased versus normal state, which can lead to better therapeutic control over an intended biological response and in the meantime offer reduced side effects. Both the basic biophysical principles underlying multivalency/multispecificity and how they influence protein design parameters were illustrated in detail in this chapter, which also included several examples regarding how these principles can be utilized to develop next-generation protein therapeutics.
After seven chapters covering many aspects of protein engineering, as described above, the next three chapters focus on DNA/RNA. Aptamers are single-stranded DNA or RNA oligonucleotides that can be selected for specific binding to a wide range of targets, primarily through the systematic evolution of ligands by exponential enrichment (SELEX) technology [11]. Chapter 15 is the first of two chapters focusing on engineering aptamers for biomedical applications, written by Dr. Li and Dr. Cao. Because of their many desirable properties such as small size, ease of synthesis, and versatile chemistry, aptamers have attracted considerable attention in many disciplines of biomedicine. After a brief introduction and description of the different strategies for selecting optimal aptamers for biomedical applications, this chapter provided a detailed overview of engineering aptamers for use in biosensors. A number of approaches were covered, which include engineering aptamers to improve the bioavailability, to generate detectable signals (e.g., through incorporation of functional nucleic acids or molecular reporters) and to achieve signal amplification for high-sensitivity/selectivity biosensing. In Chap. 16, Dr. Cerchia and co-workers provided a comprehensive summary on the development of multifunctional aptamer-based bioconjugates for targeted delivery of therapeutics and imaging agents to diseased cells and tissues. Many approaches have been utilized to conjugate aptamers with a broad array of agents, which include siRNA/miRNA, drugs, synthetic polymers, nanoparticles (e.g., synthetic nanoparticles, liposomes, gold nanoparticles, quantum dots, magnetic nanoparticles, and silica-coated nanoparticles), and radioisotopes (e.g., 99mTc for single-photon emission computed tomography imaging). Lastly, various strategies used to enhance the resistance of aptamers to nuclease degradation were also described in this chapter.
One intriguing approach for the treatment of cancer is to use vaccines. Dozens of DNA vaccines have entered clinical trials for a variety of malignancies, with demonstrated efficacy in eliciting immune responses and potential clinical responses [12, 13]. Recently, a DNA vaccine was approved for the treatment of canine melanoma, which represents a landmark achievement in this area [14]. In Chap. 17, Dr. McNeel and Dr. Olson presented an excellent overview of engineering DNA vaccines for cancer therapy. Generally speaking, a DNA vaccine for cancer is a bacterial DNA plasmid that encodes the cDNA of a tumor antigen, which can elicit humoral and/or cellular immunity against tumor cells expressing the encoded antigen when injected into recipients. A distinct advantage of DNA vaccines over other methods of antigen delivery is that DNA vaccines can be easily constructed, purified, and delivered to recipients. In this chapter, the engineering efforts to enhance the immune and anti-cancer efficacy of DNA vaccines were illustrated in extensive detail, focusing on specific modifications that can be made to the DNA backbone to enhance the expression, processing, and presentation of the encoded antigen, in addition to improve the inherent immunogenicity of the vaccine itself.
The next ten chapters, part III of this book, are related to the exciting topic of nanoengineering. Nanotechnology, an interdisciplinary research field involving physics, chemistry, engineering, biology, and medicine among others, holds tremendous potential for early detection, accurate diagnosis, and personalized treatment of diseases [15–17]. With the sizes several orders of magnitude smaller than human cells, nanoscale agents/devices can offer unprecedented interactions with biomolecules both on the surface of and inside cells, which can revolutionize disease diagnosis and treatment. In light of this, there has been numerous nanotechnology centers established worldwide over the last two decades [18–20]. One major area of applications for nanotechnology is biomedicine, and it is expected that nanotechnology will mature into a clinically useful field in the near future, which has already made significant impact in many aspects to date.
In Chap. 18, Dr. Seto and Dr. Conroy gave an excellent overview of the multifunctional nanoscale delivery systems for nucleic acids. Although nanoscale systems are attractive platforms for in vivo delivery of nucleic acids, their potential for improving human health has yet to be fully realized in the clinic. Engineering efforts is critical for modifying and optimizing synthetic and viral delivery systems to include drugs, imaging agents, and targeting moieties, which can simultaneously minimize toxicity and increase delivery efficiency/specificity. This chapter focuses on the recent advances in RNA/DNA delivery with an emphasis on the progress toward human therapies, the challenges that have been encountered, and the engineering approaches that have been employed. The delivery systems used (e.g., viral, non-viral, and directed delivery) and the therapeutic components incorporated (e.g., DNA, RNA, and nucleic acid analogs) were all described in exquisite detail. Lastly, three major challenges were identified, namely the development of optimal systems for preclinical testing, integration of clinically useful imaging approaches, and rapid translation of directed delivery technologies, which should all be adequately addressed before broad future clinical applications.
In Chap. 19, Dr. Chang and co-workers centered on engineering nanomaterials for biosensing and therapeutic applications, which provided a timely overview on the synthetic methodology, surface engineering, physiological itinerary, and theranostic applications for various inorganic and organic nanostructures. After an introduction and a brief review of the applications and preparative methods of inorganic nanomaterials, the effects of surface modification with polymers and morphology on the physiological itinerary and toxicity of these nanomaterials were discussed, which can offer critical insights for future design of targeted imaging/therapeutic agents. Subsequently, in this chapter, several examples of promising nanotechnologies and theranostic applications were given, such as biosensors, magnetic hyperthermia, and photodynamic therapy for diagnosis and treatment of cancer. To take full advantage of these innovative ideas and demonstrated proof of principles of nanomaterials for biomedical applications, it is crucial to emphasize and expand efforts on translational research and development in the near future.
The following five chapters in part III of this book are related to several classes of nanomaterials that have been extensively investigated over the last decade, all of which hold tremendous potential for clinical applications: fluorescent nanoparticles (Chap. 20), magnetic nanoparticles (Chap. 21), upconversion nanoparticles (Chap. 22), mesoporous silica nanoparticles (Chap. 23), and carbon nanomaterials (Chap. 24). Fluorescent nanoparticles have been extensively studied in preclinical research for cancer imaging and/or theranostic applications [21, 22]. In Chap. 20, Dr. Chen and Dr. Silvestre offered in-depth discussions about the design of fluorescent nanoparticles, which has to take into consideration not only the parameters that are related to enhanced fluorescence (e.g., absorption coefficient, quantum yield, stability, and excitation/emission wavelengths), but also characteristics that can lead to optimal blood circulation half-life, tumor specificity, efficient delivery, biocompatibility, and low toxicity. Three major classes of fluorescent nanoparticles were discussed: quantum dots and fluorescent dye-loaded inorganic and organic nanoparticles (e.g., those that are based on calcium phosphate, silica, lipid, or polymer). The characteristics, advantages, limitations, and the engineering strategies employed to enhance their in vivo use were discussed for each class of nanoparticles with ample preclinical examples. Significant emphasis was also devoted to clinical translation, which has remained a major challenge for most nanoparticles, and a few examples as well as the hurdles that exist were illustrated and discussed.
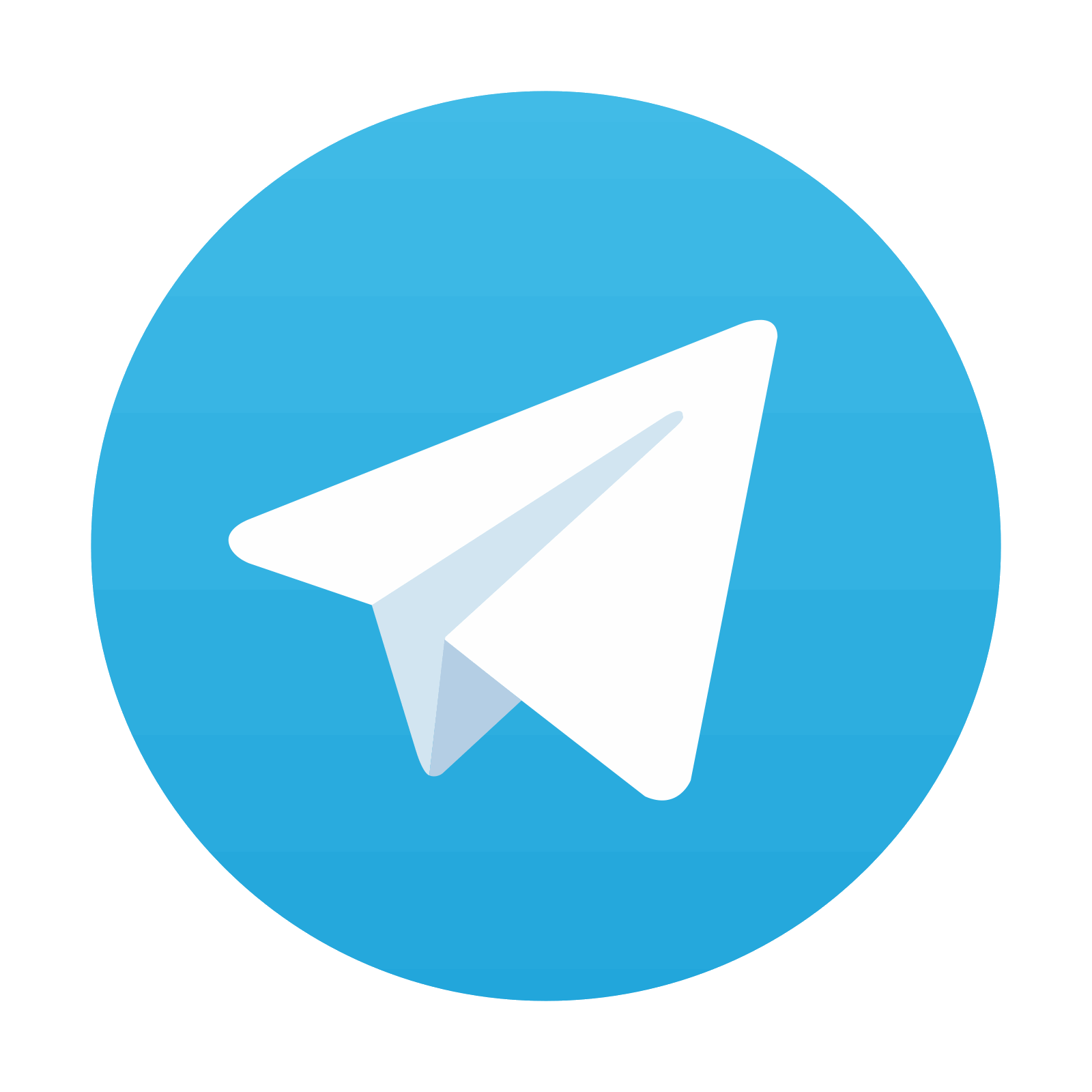
Stay updated, free articles. Join our Telegram channel
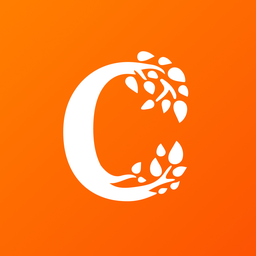
Full access? Get Clinical Tree
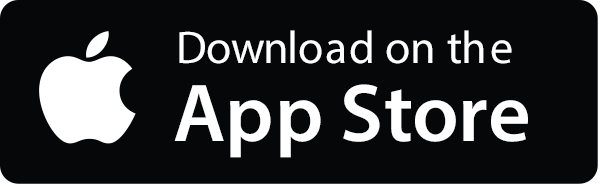
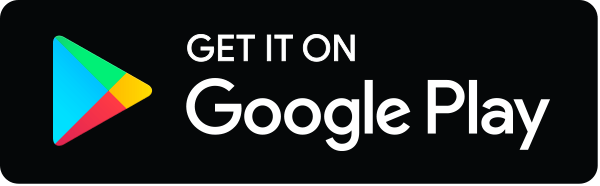