Fig. 2.1
DNA structure. Schematic representation of a DNA double helix unwound to show the complementarity of bases and the antiparallel structure of the phosphate (P) and sugar (S) backbone strands
DNA Synthesis
The synthesis of a new molecule of DNA is called replication. This process requires many enzymes and cofactors. The first step of the process involves breakage of the hydrogen bonds that hold the DNA strands together. DNA helicases and single-strand binding proteins work to separate the strands and keep the DNA exposed at many points along the length of the helix during replication. The area of DNA at the active region of separation is a Y-shaped structure referred to as a replication fork. These replication forks originate at structures called replication bubbles, which, in turn, are at DNA sequences called replication origins. The molecular sequence of the replication origins has not been completely characterized. Replication takes place on both strands, but nucleotides can only be added to the 3′ end of an existing strand. The separated strands of DNA serve as templates for production of complementary strands of DNA following Chargaff’s rules of base pairing.
The process of DNA synthesis differs for the two strands of DNA because of its antiparallel structure. Replication is straightforward on the leading strand. The enzyme DNA polymerase I facilitates the addition of complementary nucleotides to the 3′ end of a newly forming strand of DNA. In order to add further nucleotides, DNA polymerase I requires the 3′-hydroxyl end of a base-paired strand.
DNA synthesis on the lagging strand is accomplished by the formation of small segments of nucleotides called Okazaki fragments [4]. After separation of the strands, the enzyme DNA primase uses ribonucleotides to form a ribonucleic acid primer.
The structure of ribonucleic acid (RNA) is similar to that of DNA, except that each nucleotide in RNA has a ribose sugar instead of deoxyribose and the pyrimidine thymine is replaced by another pyrimidine, uracil (U). RNA also differs from DNA in that it is a single-stranded molecule. This RNA primer is at the beginning of each Okazaki segment to be copied, provides a 3′-hydroxyl group, and is important for the efficiency of the replication process. The ribonucleic acid primer then attracts DNA polymerase I. DNA polymerase I brings in the nucleotides and also removes the RNA primer and any mismatches that occur during the process. Okazaki fragments are later joined by the enzyme DNA ligase. The process of replication is semiconservative because the net result is creation of two identical DNA molecules, each consisting of a parent DNA strand and a newly synthesized DNA strand. The new DNA molecule grows as hydrogen bonds form between the complementary bases (Fig. 2.2).


Fig. 2.2
Semiconservative replication. Complementary nucleotides are added directly to the 3′ end of the leading strand, whereas the lagging strand is copied by the formation of Okazaki fragments
Protein Synthesis
The genetic information of DNA is stored as a code; a linear sequence of nitrogenous bases in triplets. These triplets code for specific amino acids that are subsequently linked together to form protein molecules. The process of protein synthesis involves several types of ribonucleic acid.
The first step in protein synthesis is transcription. During this process, DNA is copied into a complementary piece of messenger RNA (mRNA). Transcription is controlled by the enzyme RNA polymerase, which functions to link ribonucleotides together in a sequence complementary to the DNA template strand. The attachment of RNA polymerase to a promoter region, a specific sequence of bases that varies from gene to gene, starts transcription. RNA polymerase moves off the template strand at a termination sequence to complete the synthesis of an mRNA molecule (Fig. 2.3).


Fig. 2.3
Transcription. A DNA molecule is copied into mRNA with the help of RNA polymerase
Messenger RNA is modified at this point by the removal of introns—segments of DNA that do not code for an mRNA product. In addition, some nucleotides are removed from the 3′ end of the molecule, and a string of adenine nucleotides are added. This poly(A) tail helps in the transport of mRNA molecules to the cytoplasm. Another modification is the addition of a cap to the 5′ end of the mRNA, which serves to aid in attachment of the mRNA to the ribosome during translation. These alterations to mRNA are referred to as mRNA processing (Fig. 2.4). At this point, mRNA, carrying the information necessary to synthesize a specific protein, is transferred from the nucleus into the cytoplasm of the cell, where it then associates with ribosomes. Ribosomes, composed of ribosomal RNA (rRNA) and protein, are the site of protein synthesis. Ribosomes consist of two subunits that come together with mRNA to read the coded instructions on the mRNA molecule.


Fig. 2.4
Messenger RNA processing. The transcribed strand of DNA is modified to produce a mature mRNA transcript
The next step in protein synthesis is translation. A chain of amino acids is synthesized during translation by using the newly transcribed mRNA molecule as a template, with the help of a third ribonucleic acid, transfer RNA (tRNA). Leder and Nirenberg and Khorana determined that three nitrogen bases on an mRNA molecule constitute a codon [5, 6]. With four nitrogenous bases, there are 64 possible three-base codons. Sixty-one of these code for specific amino acids, and the other three are “stop” codons that signal the termination of protein synthesis. There are only 20 amino acids, but 61 codons. Therefore, most amino acids are coded for by more than one mRNA codon. This redundancy in the genetic code is referred to as degeneracy.
Transfer RNA molecules contain “anticodons”—nucleotide triplets that are complementary to the codons on mRNA. Each tRNA molecule has attached to it the specific amino acid for which it codes.
Ribosomes read mRNA one codon at a time. Transfer RNA molecules transfer the specific amino acids to the synthesizing protein chain (Fig. 2.5). The amino acids are joined to this chain by peptide bonds. This process is continued until a stop codon is reached. The new protein molecule is then released into the cell milieu and the ribosomes split apart (Fig. 2.6).



Fig. 2.5
Translation. Transfer RNA molecules bring in specific amino acids according to the triplet codon instructions of mRNA that are read at the ribosomes

Fig. 2.6
Overview of protein synthesis. DNA is transcribed to mRNA, which is modified to mature transcript and then transferred to the cytoplasm of the cell. The codons are read at the ribosomes and translated with the help of tRNA. The chain of amino acids produced during translation is joined by peptide bonds to form a protein molecule
DNA Organization
Human chromatin consists of a single continuous molecule of DNA complexed with histone and nonhistone proteins. The DNA in a single human diploid cell, if stretched out, would be approximately 2 m in length and therefore must be condensed considerably to fit within the cell nucleus [7]. There are several levels of DNA organization that allow for this.
The DNA helix itself is the first level of condensation. Next, two molecules of each of the histones H2A, H2B, H3, and H4 form a protein core: the octamer. The DNA double helix winds twice around the octamer to form a 10-nm nucleosome, the basic structural unit of chromatin. Adjacent nucleosomes are pulled together by a linker segment of the histone H1. Repeated, this gives the chromatin the appearance of “beads on a string.” Nucleosomes are further coiled into a 30-nm solenoid, with each turn of the solenoid containing about six nucleosomes. The solenoids are packed into DNA looped domains attached to a nonhistone protein matrix. Attachment points of each loop are fixed along the DNA. The looped domains coil further to give rise to highly compacted units, the chromosomes, which are visible with the light microscope only during cell division. Chromosomes reach their greatest extent of condensation during mitotic metaphase (Fig. 2.7).
Chromosome Structure
A chromosome consists of two sister chromatids, each of which is comprised of a contracted and compacted double helix of DNA. The centromere, telomere, and nucleolar organizer regions are functionally differentiated areas of the chromosomes (Fig. 2.8).


Fig. 2.8
The functional and structural components of metaphase chromosomes
The Centromere
The centromere is a constriction visible on metaphase chromosomes where the two sister chromatids are joined together. The centromere is essential to the survival of a chromosome during cell division. Interaction with the mitotic spindle during cell division occurs at the centromeric region. Mitotic spindle fibers are the functional elements that separate the sister chromatids during cell division.
Human chromosomes are classified based on the position of the centromere on the chromosome. The centromere is located near the middle in metacentric chromosomes, near one end in acrocentric chromosomes, and between the middle and end in submetacentric chromosomes. The kinetochore apparatus is a complex structure consisting of proteins that function at the molecular level to attach the chromosomes to the spindle fibers during cell division. Although the kinetochore is located in the region of the centromere, it should not be confused with the centromere. The latter is the DNA at the site of the spindle-fiber attachment.
The Nucleolar Organizer Regions
The satellite stalks of human acrocentric chromosomes contain the nucleolar organizer regions (NORs), so-called because this is where nucleoli form in interphase cells. NORs are also the site of ribosomal RNA genes and production of rRNA. In humans, there are theoretically ten nucleolar organizer regions, although all may not be active during any given cell cycle.
The Telomeres
The telomeres are the physical ends of chromosomes. Telomeres act as protective caps to chromosome ends, preventing end-to-end fusion of chromosomes and DNA degradation resulting after chromosome breakage. Nonhistone proteins complex with telomeric DNA to protect the ends of chromosomes from nucleases located within the cell [9]. The telomeric region also plays a role in synapsis during meiosis. Chromosome pairing appears to be initiated in the subtelomeric regions [10].
Telomeres contain tandem repeats of the nitrogenous base sequence TTAGGG over 3–20 kb at the chromosome ends [11]. At the very tip of the chromosome, the two strands do not end at the same point, resulting in a short G-rich tail that is single stranded. Because of this, DNA synthesis breaks down at the telomeres and telomeres replicate differently than other types of linear DNA. The enzyme telomerase synthesizes new copies of the telomere TTAGGG repeat using an RNA template that is a component of the telomerase enzyme. Telomerase also counteracts the progressive shortening of chromosomes that results from many cycles of normal DNA replication. Telomere length gradually decreases with the aging process and with increased numbers of cell divisions in culture. The progressive shortening of human telomeres appears to be a tumor-suppressor mechanism [12]. The maintenance of telomeric DNA permits the binding of telomeric proteins that form the protective cap at chromosome ends and regulate telomere length [12]. Cells that have defective or unstable telomerase will exhibit shortening of chromosomes, leading to chromosome instability and cell death.
Types of DNA
DNA is classified into three general categories: unique sequence, highly repetitive sequence DNA (>105 copies), and middle repetitive sequence DNA (102–104 copies). Unique sequence or single-copy DNA is the most common class of DNA, comprising about 75% of the human genome [13]. This DNA consists of nucleotide sequences that are represented only once in a haploid set. Genes that code for proteins are single-copy DNA. Repetitive or repeated sequence DNA makes up the remaining 25% of the genome and is classified according to the number of repeats and whether the repeats are tandem or interspersed among unique sequence DNA [13].
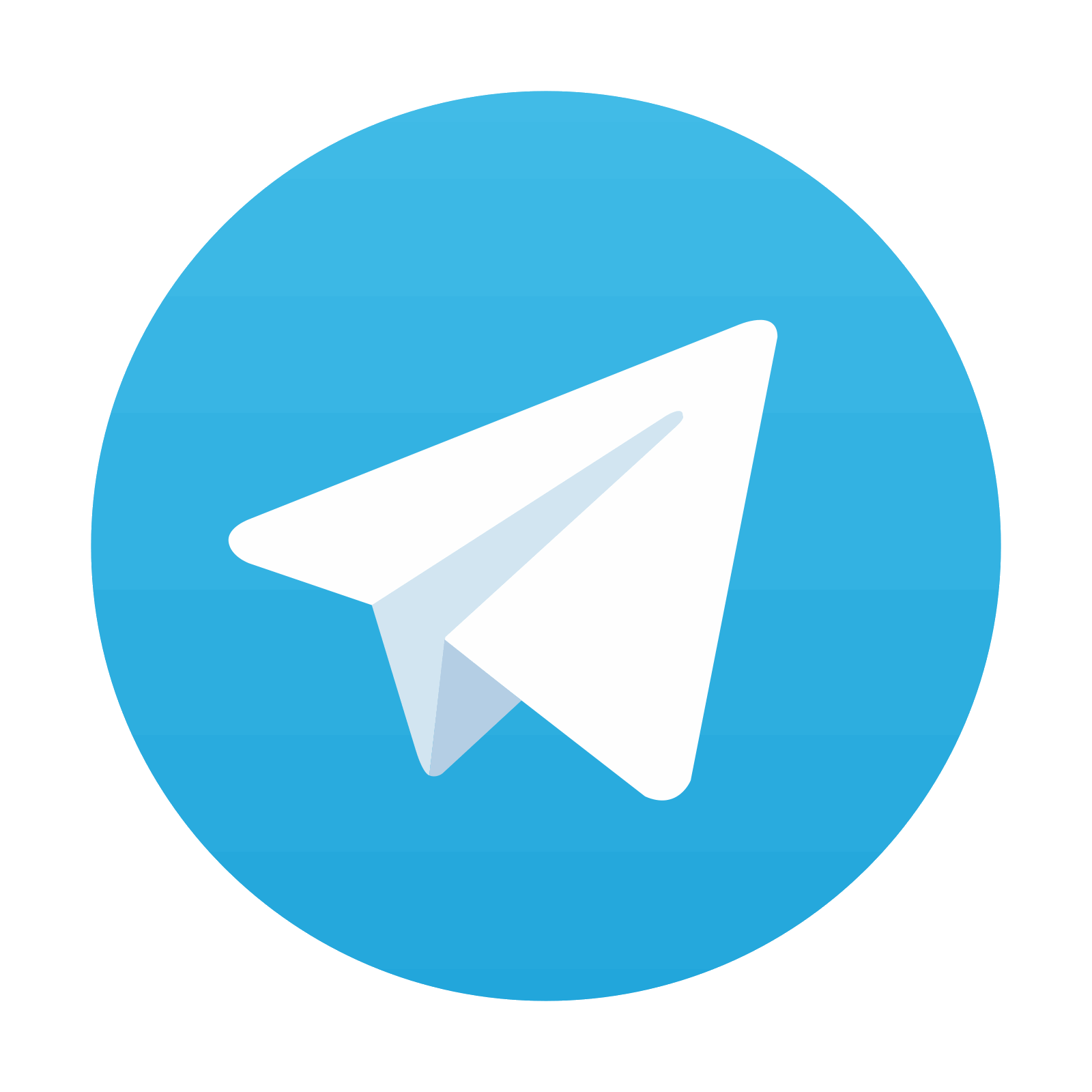
Stay updated, free articles. Join our Telegram channel
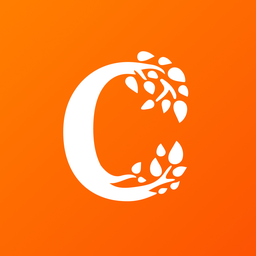
Full access? Get Clinical Tree
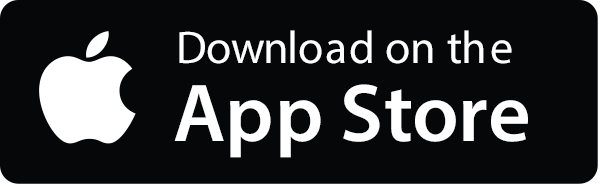
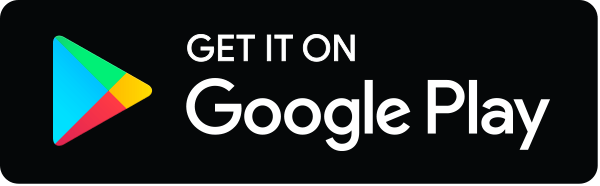