Introduction
The hypothalamus is the part of the brain where activity of the autonomic nervous system and endocrine glands, which directly control various systems of the body, is integrated with input from other centers that give rise to emotions and behavior. The hypothalamus thus serves to ensure that (1) the organism responds appropriately to deviations from various internal set points (including those for temperature, volume, osmolality, satiety, and body fat content), (2) the responses to such deviations from a set point include coordinated activity of the nervous and endocrine systems, and (3) the emotions and behavior being manifested are appropriate for reflex responses being triggered to correct the deviations from internal set points. The following description outlines the integrative function of the hypothalamus in regard to the coordination of endocrine and CNS responses.
Intravascular volume loss from any cause activates autonomic neural responses, mainly via the sympathetic nervous system to retain fluid and electrolytes, maintain blood pressure through vascular smooth muscle contraction, and maintain cardiac output by increasing heart rate. The effect of these immediate neural responses is reinforced by activation of several hormonal systems. In response to a decrease in intravascular volume, the renin-angiotensin-aldosteronesystem (RAAS) is activated and sodium is retained. Additionally increasing osmolarity triggers thirst and leads to release of vasopressin (antidiuretic hormone [ADH]) from hypothalamic neurons that end in the posterior pituitary, resulting in free water absorption in the kidney. In short, the body maintains intravascular volume by regulating sodium reabsorption through aldosterone, while it regulates osmolarity by increasing fluid intake (thirst) and free water retention by vasopressin.
Emotions interplay with these systems to coordinate appropriate behavioral and hormonal responses. Fear and pain activate limbic, hypothalamic and other centers to coordinate respective defensive (fight or flight) and recuperative stereotypic behaviors. These emotional responses to various stressors (eg, perceived threat to body; fear) also activate the sympathetic nervous system and the hypothalamic-pituitary-adrenal (HPA) axis, which coordinate the mammalian stress response through preparing the body for “fight and flight” and through mobilization of energy stores. Any kind of stress (eg, physical, mental, metabolic stress) leads to the release of corticotropin-releasing hormone (CRH) from the hypothalamus and consequent adrenocorticotropin (ACTH; pituitary) and cortisol (adrenal cortex) secretion. For example, starvation leads to the activation of the HPA axis and ultimately cortisol-mediated increased gluconeogenesis to maintain basic physiologic functions.
The pituitary gland is the partner of the hypothalamus on the body side of the mind-body interface. Once viewed as the “master gland” in regulation of neuroendocrine systems, the pituitary is now known to be a “middle manager” responding to input from both the brain (via the hypothalamus) and the body (via the various peripheral endocrine glands).
The basic framework for hypothalamic-pituitary function is the neuroendocrine axis, a cascade of interacting hormonal products from various regions of the CNS to the hypothalamus, anterior pituitary gland, peripheral endocrine end organs, and peripheral target tissues. Some neuroendocrine axes involve hormones released by the hypothalamus that stimulate cells in the anterior pituitary to secrete other hormones into the systemic circulation. Each of these anterior pituitary hormones travels to a distant endocrine gland to stimulate secretion of yet other hormones that affect various target tissues. Thus, disorders of the hypothalamus and pituitary have important consequences for the pathophysiologic mechanisms of a wide range of disorders involving many different tissues and organs.
This chapter focuses on five clinical entities. The first four reflect the diversity of pituitary disease: pituitary adenomas, panhypopituitarism, vasopressin excess, and vasopressin deficiency. The last, obesity, is one in which the hypothalamus plays a crucial role and which has enormous implications for diseases involving many other organ systems.
Normal Structure & Function of the Hypothalamus & Pituitary Gland
The hypothalamus is located in the floor and lateral walls of the third ventricle below the hypothalamic sulcus and comprises about 1% of the mass of the brain (Figure 19–1). Hypothalamic nuclei are clusters of neurons whose cell bodies lie in discrete regions (Figure 19–2). From these nuclei, hypothalamic neurons send projections either directly or via neuronal relay to other parts of the central and peripheral nervous systems and secrete hormones that make possible the hierarchical control of various physiologic processes (Table 19–1).
Nucleus | Location | Major Neurohormones and/or Functions |
---|---|---|
Supraoptic (SON) | Anterolateral, above the optic tract |
|
Paraventricular (PVN) | Dorsal anterior periventricular |
|
Suprachiasmatic (SCN) | Above the optic chiasm, anteroventral periventricular zone |
|
Arcuate (ARCN) | Medial basal hypothalamus close to the third ventricle |
|
Periventricular | Anteroventral |
|
Ventromedial (VMN) |
| |
Dorsomedial (DMN) |
| |
Lateral hypothalamus |
| |
Preoptic area (POA) |
| |
Anterior hypothalamus |
| |
Posterior hypothalamus |
|
The hypothalamus is connected to the pituitary gland by a stalk, which is composed of axons of some hypothalamic neurons with terminal boutons comprising the posterior pituitary gland (Figure 19–3). The posterior pituitary neurons secrete the peptide hormones oxytocin and vasopressin directly into the systemic circulation. The development of the anterior pituitary from the oral ectoderm is dictated by a tight program of consecutive activation of distinct transcription factors in the differentiating pituitary cell types (Figure 19–4). The pituitary gland is encased in a tough fibrous capsule, positioned in the bony sella turcica. The pituitary gland is bounded above by the optic chiasm and laterally by the cavernous sinus and the structures that traverse it (internal carotid artery, cranial nerves [CN] III and IV, first and second divisions of CN V and VI).
Figure 19–3
The component parts of the pituitary and their relationship to the hypothalamus. The pars tuberalis, pars distalis, and pars intermedia, which are rudimentary in humans, form the adenohypophysis. The infundibulum and pars nervosa form the neurohypophysis. (Redrawn and modified, with permission, from the Ciba Collection of Medical Illustrations, by Frank H. Netter, MD.)
Figure 19–4
Diagram of the transcription factors involved in anterior pituitary development. Factors on the left are mainly responsible for Rathke pouch formation and early pituitary development. On the right side are factors inducing the differentiation into the major five pituitary cell types. Mutations of some of the genes encoding these transcription factors have been shown to result in hypopituitarism.
In circumventricular parts of the CNS, the capillaries are fenestrated, allowing neurons to sense various specific chemical stimuli in the bloodstream. These sensory neurons transmit the information regarding changes in stimuli (eg, change in osmolality) to other hypothalamic neurons involved in a variety of specific types of secretory activities.
Other hypothalamic neurons secrete peptide hormones into a specialized capillary bed termed the pituitary portal system. Blood in this capillary system flows directly from the median eminence to the anterior pituitary gland, where specific cells that display receptors for the various hypothalamic releasing hormones are found. Binding of hypothalamic hormones to their receptors on cells of the anterior pituitary in turn stimulates the secretion of specific anterior pituitary hormones into the systemic circulation. The portal system allows the cells of the anterior pituitary to be bathed in blood rich in hypothalamic hormones without the dilution that would have occurred in the systemic circulation. This intimate connection between hypothalamus and pituitary has important pathophysiologic consequences (see later).
Once secreted, the anterior pituitary hormones travel via the general bloodstream throughout the body and trigger the release of other hormones from particular endocrine glands. These hormones, in turn, have effects on target tissues that influence growth, reproduction, metabolism, and responses to stress. In addition to their effects on target tissues, hormones secreted in response to stimulation by pituitary hormones also feed back and inhibit secretion of the corresponding pituitary and hypothalamic hormones.
The posterior pituitary hormones are involved in a very different type of neuroendocrine axis, one that bypasses secondary endocrine glands and affects peripheral target tissues directly.
Although most peptide factors secreted by the hypothalamus cause release of a pituitary hormone, some are inhibitory factors that block or diminish secretion of particular hormones. There are five main cell types in the anterior pituitary, each of which produces and secretes one of five families of hormones: pro-opiomelanocortin and adrenocorticotropic hormone (ACTH), thyrotropin (TSH), growth hormone (GH), prolactin (PRL), and the gonadotropins, luteinizing hormone (LH), and follicle-stimulating hormone (FSH) (Table 19–2).
ACTH | GH | Prolactin | TSH | LH | FSH | |
---|---|---|---|---|---|---|
Peptides | Derived from POMC precursor | Single-chain polypeptide | Single-chain polypeptide | α: α1 | α: α1 | α: α1 |
β: TSH-β | β: LH-β | β: FSH-β | ||||
Receptor | ACTH-receptor (melanocortin-2-receptor) | GH receptor | Prolactin receptor | TSH receptor | LH receptor | FSH receptor |
Source | Corticotropes (pituitary) | Somatotropes (pituitary) | Lactotropes (pituitary) | Thyrotropes (pituitary) | Gonadotropes (pituitary) | Gonadotropes (pituitary) |
Hypothalamic releasing hormone | CRH, AVP | GHRH (ghrelin) | TRH | TRH | GnRH | GnRH |
Hypothalamic inhibiting factors | Somatostatin | Dopamine | Somatostatin, dopamine | |||
Target | Adrenal gland | Liver (production of IGF-1), peripheral tissue | Mammary gland | Thyroid gland | Ovary (theca cell, granulosa cell, luteal cell)/testis (Leydig cell) | Ovary (granulosa cell)/testis (Sertoli cell) |
Function | Stimulating cortisol and adrenal androgen release | Stimulating growth (direct and indirect effect via IGF-1) | Stimulating lactation | Stimulating thyroid hormone release | Stimulating estrogen/testosterone production | Regulating granulosa and Sertoli cell function |
In addition to their roles in regulation of neuroendocrine axes, some hypothalamic and pituitary hormones are important, but poorly understood, regulators of immune functions and the inflammatory response. Furthermore, secretion of hypothalamic and pituitary hormones can be significantly influenced by cytokines that regulate the immune response.
Checkpoint
What is the role of the hypothalamus?
What are the neuroendocrine axes, and how do they work?
What structures surround the pituitary?
Where do the neurons whose axons comprise the posterior pituitary originate?
Physiology of the Hypothalamus & Pituitary Gland
The HPA axis is a major part of the physiologic stress system. A variety of stressors (eg, metabolic, physical, mental stress) result in activation of the HPA axis. The major hypothalamic regulator is the peptide CRH and to a lesser extent arginine vasopressin (AVP), which are produced in the paraventricular and supraoptic nuclei of the hypothalamus and are released into the hypothalamic-pituitary portal system. These hormones trigger synthesis and intracellular transport of a large protein termed pro-opiomelanocortin (POMC). POMC is further processed by proteases (prohormone convertases) to release smaller peptides, including a 39-amino-acid-residue peptide, ACTH (Figure 19–5). Although ACTH is the major pituitary hormone that stimulates adrenocortical endocrine function, the amino-terminal part of the POMC peptide (N-POMC) seems to harbor an adrenal growth-promoting function.
Figure 19–5
Schematic representation of the prepro-opiomelanocortin molecule formed in pituitary cells, neurons, and other tissues. The numbers in parentheses identify the amino acid sequences in each of the polypeptide fragments. For convenience, the amino acid sequences are numbered from the amino terminal of corticotrope (ACTH) and read toward the carboxyl terminal portion of the parent molecule, whereas the amino acid sequences in the other portion of the molecule read to the left to –131, the amino terminal of the parent molecule. The locations of Lys-Arg and other pairs of basic amino acid residues are also indicated; these are the sites of proteolytic cleavage in the formation of the smaller fragments of the parent molecule. AL, anterior lobe; IL, intermediate lobe. (Redrawn, with permission, from Barrett KE et al, eds. Ganong’s Review of Medical Physiology, 24th ed. McGraw-Hill, 2011.)
ACTH released into the systemic circulation triggers synthesis and secretion of corticosteroids and adrenal androgens. The effect of ACTH on mineralocorticoid synthesis and release is much less pronounced, as it is mainly regulated by the RAAS.
These steroid hormones, in turn, have complex effects on many tissues to protect the individual from stress: They raise blood pressure and blood glucose, alter responsiveness of the immune system, and so on. Glucocorticoids also feed back to the hypothalamus, where they inhibit CRH secretion, and to the pituitary, where they further inhibit ACTH secretion. In the absence of unusual stress, there is a daily diurnal rhythm of CRH, ACTH, and adrenal steroid release.
Pituitary factors (ie, N-POMC, ACTH) are involved in regulating proliferation of adrenal cells and growth of the adrenal layers involved in glucocorticoid and androgen secretion. As a result of chronic activation of the HPA axis, hypertrophy of the target organ (adrenal cortex) occurs. Conversely, conditions that downregulate the HPA axis (eg, exogenous glucocorticoids) result in atrophy of the adrenal cortex. On the other hand, the overall tone of the HPA axis has little or no effect on the growth of the mineralocorticoid-secreting tissues, despite the fact that acute ACTH stimulation triggers the release of mineralocorticoids.
TSH and the gonadotropins belong to the family of glycoprotein hormones (Table 19–2). The classic glycoprotein hormone family members TSH and the gonadotropins, FSH and LH, as well as the placenta-derived pregnancy hormone human chorionic gonadotropin (hCG) are composed of a common α-glycoprotein subunit (α-GSU) and an individual β-subunit (eg, TSH-β, LH-β). The unique β-subunit of the glycoprotein hormones is responsible for the biologic differences of these hormones. Another member of this family is thyrostimulin, which shares the composition of an α- and β-subunit (α-2, β-5). The physiologic role of this hormone has yet to be determined.
Thyrotropin (thyroid-stimulating hormone [TSH]) is released from specific cells in the pituitary on stimulation by thyrotropin-releasing hormone (TRH) from the hypothalamus. A hypothalamic factor negatively regulating TSH release is somatostatin. TSH, in turn, travels via the systemic bloodstream to the thyroid gland, where it stimulates synthesis and secretion of the thyroid hormones thyroxine and triiodothyronine. Thyroid hormones have effects on nearly every tissue in the body but especially the cardiovascular, respiratory, skeletal, and central nervous systems. Thyroid hormones are critical at key points in development, and their deficiency during development has effects (eg, severe mental retardation and short stature) that are not fully reversible by subsequent thyroid hormone administration (Chapter 20).
Besides its target tissue effects, thyroid hormone feeds back to the pituitary and hypothalamus to inhibit secretion of TSH and TRH. TSH also triggers growth of thyroid tissue, resulting in goiter under conditions of chronic TSH stimulation such as iodine deficiency (see Chapter 20).
The role of the gonadotropins is to regulate the reproductive system’s neuroendocrine axis. Thus, a releasing factor from the hypothalamus termed gonadotropin-releasing hormone (GnRH) stimulates LH and FSH secretion, which stimulates steroidogenesis within the ovaries and testes. Furthermore, the gonadotropins promote Sertoli and theca cell function and gametogenesis. The steroids produced by the ovaries (estrogens) and by the testes (testosterone) inhibit GnRH, LH, and FSH production and have target tissue effects on developing follicles within the ovary itself, on the uterus (controlling the menstrual cycle), on breast development, on spermatogenesis, and on many other tissues and physiologic processes (see Chapters 22 and 23).
As is the case with all neuroendocrine axes, the simple feedback loop is complicated by other inputs (eg, from the CNS) that modify responsiveness (Chapter 7). The discovery that KiSS1-derived peptides (eg, metastin) induce hypothalamic GnRH-release via signaling through a G protein–coupled receptor (GPR54) illustrates this point. Mutations in either of these can result in the failure of puberty to develop. A notable feature for many hypothalamic releasing factors, but particularly GnRH, is that secretion occurs in pulsatile fashion and that changes in the rate and amplitude of secretion result in altered pituitary responsiveness because of downregulation or upregulation of the receptors for the hypothalamic releasing factors found on the surface of the pituitary cells. Not only is the secretion of GnRH episodic, but the secretion of FSH and LH is as well, with a secretory burst every 60 minutes. The gonadotropins follow a typical secretion pattern during the estrus cycle with a midcycle LH surge initiating ovulation.
Growth hormone and prolactin are structurally related single-chain polypeptides with different spectrums of action.
Growth hormone (GH), positively regulated by hypothalamic growth hormone–releasing hormone (GHRH) and inhibited by somatostatin, triggers growth-promoting effects in a wide range of tissues (Figure 19–6). GH has direct (eg, stimulating the growth of cartilage) as well as indirect (eg, via insulin-like growth factor-1 [IGF-1], a polypeptide secreted by the liver and other tissues) actions (Figure 19–7). IGF-1 has insulin-like effects of promoting fuel storage in various tissues. IGF-1 in turn inhibits GHRH and GH secretion. As in the other neuroendocrine feedback axes, the CNS and other factors can significantly influence the simple regulatory axis (Table 19–3). One of these factors is the gastrointestinal peptide hormone, ghrelin, which acts through the growth hormone–secretagogue receptor to induce GH release. The physiological significance of this process has yet to be determined. Somatostatin inhibits GH release and somatostatin analogs are therefore used to inhibit GH secretion from GH-secreting pituitary tumors.
Figure 19–6
Schematic diagram of the hypothalamic control of growth hormone secretion. Inhibitory arrows are dashed; stimulating arrows are solid. GHRH, growth hormone–releasing hormone; IGF, insulin-like growth factor. (Redrawn from Reichlin S. Neuroendocrinology. In: Wilson JD et al, eds. Williams Textbook of Endocrinology, 9th ed. Saunders, 1998.)
Figure 19–7
Schematic representation of multiple sites of growth hormone (GH) action. GHRH, growth hormone–releasing hormone; GHRP, growth hormone–releasing peptide; IGF-I, insulin-like growth factor I. (Redrawn, with permission, from Thorner MO et al. The anterior pituitary. In: Wilson JD et al, eds. Williams Textbook of Endocrinology, 9th ed. Saunders, 1998.)
Factor | Augmented Secretion | Inhibited Secretion |
---|---|---|
Neurogenic | Stage III and stage IV sleep | REM sleep |
Stress (traumatic, surgical, inflammatory, psychic) | Alpha-adrenergic antagonists | |
Alpha-adrenergic agonists | Beta-adrenergic agonists | |
Beta-adrenergic antagonists | Acetylcholine antagonists | |
Dopamine agonists | ||
Acetylcholine agonists | ||
Metabolic | Hypoglycemia | Hyperglycemia |
Fasting | Rising fatty acid level | |
Falling fatty acid level | Obesity | |
Amino acids | ||
Uncontrolled diabetes mellitus | ||
Uremia | ||
Hepatic cirrhosis | ||
Hormonal | GHRH | Somatostatin |
Low insulin-like growth factor | High insulin-like growth factor | |
Estrogens | Hypothyroidism | |
Glucagon | High glucocorticoid levels | |
Arginine vasopressin | ||
Ghrelin |
Some of the actions of GH appear to have a counter-regulatory character in that they raise blood glucose levels and antagonize the action of insulin. In contrast, other actions of GH via IGF-1 are insulin-like. This apparent contradiction makes sense when one considers that promoting growth requires first raising blood levels of substrates and then using them for synthesis. To do the latter without the former would simply make the individual hypoglycemic without promoting long-term growth.
The primary role of prolactin in humans is to stimulate breast development and milk synthesis. It is discussed in greater detail in Chapter 22. Prolactin secretion is mainly negatively regulated by the neurotransmitter dopamine from the hypothalamus rather than by a peptide. That is, dopamine acts to inhibit rather than stimulate prolactin secretion. Pathologic processes that result in separation of the pituitary gland from the hypothalamus cause loss of all pituitary hormones except prolactin (panhypopituitarism from lack of the hypothalamic releasing hormones). Loss of dopamine results instead in an increase in prolactin secretion from specific anterior pituitary cells now freed of inhibition by dopamine. Primary hypothyroidism is often accompanied by hyperprolactinemia because increased levels of TRH can exhibit prolactin-releasing factor properties.
The peptide hormones vasopressin and oxytocin are synthesized in the supraoptic and paraventricular nuclei of the hypothalamus. The axons of the neurons in these nuclei form the posterior pituitary, where these peptide hormones are stored. Thus, there is no need for a separate set of hypothalamic releasing factors to trigger vasopressin or oxytocin release.
In response to a small increase in blood osmolality, the hypothalamic “osmostat” responds by triggering the subjective sense of thirst and at the same time the release of vasopressin. Vasopressin increases the number of active water channels in the cell membranes of renal collecting duct cells, allowing conservation of free water. This increases the concentration of the urine. Conservation of free water and stimulation of thirst have the net effect of correcting the small change in blood osmolality.
Vasopressin binds to at least three classes of receptors. One of these classes of vasopressin receptors (V1A) is found on smooth muscle. Its major effect is to trigger vasoconstriction. V1B receptors are found on corticotropes, and they contribute to increased ACTH secretion. The other class of receptors (V2) is found in the distal nephrons in the kidneys; its major action is to mediate vasopressin’s effects on osmolality. Because of its V2-mediated actions, vasopressin is also known as antidiuretic hormone (ADH). The relationship among osmotic forces, volume, and vasopressin secretion is illustrated in Figure 19–8. Although the minute-to-minute function of vasopressin is to maintain blood osmolality, its secretion is also increased by large decreases in intravascular volume. This assists aldosterone in raising intravascular volume, albeit at the expense of lowered osmolality. The combination of ADH-mediated peripheral vasoconstriction and water retention (in the setting of hypotension even with lower or normal osmolarity) can be understood as a way of helping to maintain perfusion in the face of major intravascular volume deficits (eg, with hemorrhage), even if the volume and osmolar composition of the perfusing blood are not ideal. In pharmacologic doses, vasopressin can be used as an adjunct in the treatment of severe hypotensive crises.
Figure 19–8
The influence of hemodynamic status on the osmoregulation of vasopressin in otherwise healthy humans. The numbers in the center circles refer to the percentage change in volume or pressure; N refers to the normovolemic normotensive subject. Note that the hemodynamic status affects both the slope of the relationship between the plasma vasopressin and osmolality and the osmotic threshold for vasopressin release. (Redrawn and adapted from Robertson GL et al. The osmoregulation of vasopressin. Kidney Int. 1976;10:25. Adapted by Rose BD in: Clinical Physiology of Acid-Base and Electrolyte Disorders, 3rd ed. McGraw-Hill, 1989. Reprinted, with permission, from Kidney International.)
Like vasopressin, this peptide is stored in nerve terminals of hypothalamic neurons in the posterior pituitary. It plays an important role in breast and uterine smooth muscle contraction both on a minute-to-minute basis during breast-feeding and in contraction of the uterus during parturition. Besides its function in parturition and lactation, recent research suggests a significant role for oxytocin in the neuropsychological regulation of behavior, such as trust formation and interpersonal bonding (eg, pair and parental attachment).
Checkpoint
How do neuroendocrine feedback loops of the anterior and posterior pituitary differ?
How can two polypeptide hormones whose mature forms have no sequence in common be derived from the same precursor?
Describe the distinguishing features of each pituitary neuroendocrine feedback axis.
What is the significance of receptor downregulation for hypothalamic control of pituitary function?
Physiology of the Neuroendocrine Axis
A number of features of neuroendocrine axis physiology have important implications for the pathophysiology of disease.
First, the hypothalamic hormones that traverse the pituitary portal system are short-lived. They also have relatively low affinities for their receptors. These properties are generally more characteristic of neurotransmitters in the nervous system than of hormones in the bloodstream. Some of these hormones, and the receptor systems with which they interact, have evolved in ways that take advantage of the unique features of a neuroendocrine axis. For example, in the case of GnRH, secretion is markedly pulsatile in character; a particular rate and amplitude of hypothalamic hormone secretion are crucial for a proper response by the receptor-bearing gonadotropes. If the pulse rate or amplitude is too high, the receptors are downregulated.
Second, for some of the neuroendocrine axes, measurement of a random blood level of the end-organ hormone is not generally clinically useful. A more reliable approach to assessment of neuroendocrine axis function is often to assess the secretory response to a provocative stimulus, or challenge test. Thus, an adequate increase in blood cortisol 1 hour after an intravenous injection of ACTH provides far more compelling evidence for an intact adrenal gland than does a randomly drawn, unprovoked normal blood level of cortisol.
Finally, besides stimulating end-organ hormone secretion, most of the pituitary hormones exert trophic effects on the hormone-secreting cells of the end organ. Thus, excess of pituitary hormone results in end-organ hypertrophy, and lack of the pituitary hormone results in end-organ atrophy.
Various physiologic control mechanisms integrated by the hypothalamus work to maintain body weight over the short and the long term (Figure 19–9).
The key parameters of short-term regulation of body weight are (1) the amount and composition of food, (2) nutrient absorption and assimilation, and (3) satiety, the sense of having eaten enough food. Satiety is a complex response to food intake that has mechanical, neural, and hormonal components.
A main mechanism by which short-term food intake and satiety are regulated is the communication via the “gut-brain axis.” The gut-brain crosstalk uses two main routes of communication, including both neural components, mainly afferent vagal fibers, and hormonal components. Thus, we feel a sense of fullness in response to mechanical distention of the stomach, which triggers afferent neural pathways to the hypothalamus or via brainstem centers (eg, nucleus of the solitary tract). In addition, hormones are secreted in response to food ingestion and absorption and have direct effects on the hypothalamus to induce satiety. These hormonal signals mainly include anorexigenic satiety signals, such as cholecystokinin (CCK) and glucagon-like peptide-1 (GLP-1), which are released in the gut and directly impact on gastrointestinal mobility and function, but also stimulate gastrointestinal neural signaling to the hypothalamus. Some of these hormones travel directly to the brain and bind to receptors in the hypothalamus or in areas of regulated “open” blood-brain barrier. The only known orexigenic signal arising from the gut is the peptide hormone, ghrelin, suggesting that satiety is more abundantly regulated by the gastrointestinal system than hunger.
In contrast to short-term control of body weight, long-term regulation is largely influenced by the degree of obesity. Fat cells secrete the hormone leptin in proportion to the amount of triglyceride they have stored. Thus, over the long term, excess ingestion of calories resulting in increased fat deposition triggers an increase in leptin secretion. Leptin impinges on its receptors in the hypothalamus so that the individual eats less and, therefore, assimilates fewer calories. Another response to leptin is to increase sympathetic nervous system activity so that more calories are burned.
Conversely, when caloric intake is insufficient to maintain body weight, fat is mobilized, leptin secretion decreases, and set points in the hypothalamus are changed in ways that promote food-seeking behavior, diminish sympathetic neural activity, and generally conserve calories to offset the tendency toward weight loss. As a result of this feedback loop, further decrease in body weight is resisted. It is likely that this system evolved primarily as a defense against starvation, but it also serves to defend against obesity.
How these signals are normally integrated in the hypothalamus to achieve satiety in the short term and maintain normal body weight in the long term is less clear. The arcuate nucleus of the hypothalamus is the best understood integrator of the regulation of food intake. However, several other hypothalamic nuclei appear to be involved in the control of energy homeostasis and food intake. For example, lesions in the ventromedial region result in obesity, and lesions in the lateral hypothalamus result in weight loss. One hypothesis attempting to integrate current information on the regulation of fuel homeostasis proposes different responses by the body to falling versus rising leptin concentrations, as would be seen in weight loss versus weight gain, respectively. Thus, in response to falling leptin levels, neuropeptide Y is secreted from leptin receptor–bearing cells of the arcuate nucleus in the ventromedial hypothalamus. Neuropeptide Y is believed to mediate hypothalamic responses to starvation.
Another well-described system regulating satiety and food intake within the arcuate nucleus of the hypothalamus is the POMC system. Though the hypothalamic POMC system uses the same peptides for signaling mediators as the pituitary POMC system, they are very different in POMC expression, processing, and receptors. In particular, the main receptor mediating satiety and food intake is a special subtype of melanocortin receptors (MC4-R). In the state of caloric excess, hypothalamic-derived POMC peptides such as melanocyte-stimulating hormone (α-MSH) keep these MC4-R in a tonic activated state. In addition, hypothalamic POMC neurons are leptin responsive; therefore, these neurons represent an interface between the leptin and the POMC system. As circulating leptin levels parallel the total quantity of fat storage, it makes sense that activation of POMC neurons leads to inhibition of food intake. In the event of caloric restriction, the tonic activation of the MC4-R is reduced by two mechanisms: a decrease in agonists (MSH) and, even more important, an increase in availability of antagonists, namely agouti-related peptide (AGRP). These antagonists downregulate not only MSH (agonist driven) but also an intrinsic constitutive activity of the MC4-R. The mode of action of the MC4-R antagonism by AGRP has been termed “inverse agonism.” Furthermore, it is believed that many other neuropeptides, including bombesin, insulin, and a group of peptides termed orexins, have complex effects on the hypothalamus that affect feeding, satiety, energy balance, and other parameters relevant for weight control (Table 19–4). The orexins appear to be ligands for previously “orphan” G protein–coupled receptors in the brain. How the effects of these peptides are integrated with those of leptin and neuropeptide Y is a current focus of research. Finally, research strongly implicates leptin in other physiologic functions such as regulating reproductive and immune function as well as bone density.
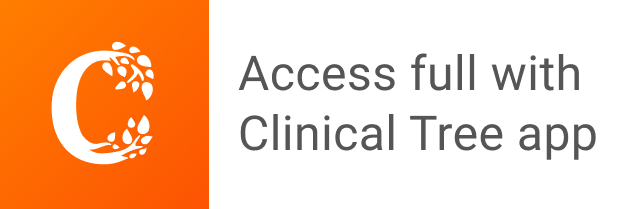