, Joseph M. Scandura1, Shahin Rafii1 and Venkat R. Pulijaal2
(1)
Department of Medicine, Weill Cornell Medical College, 1300 York Avenue, New York, NY 10064, USA
(2)
Pathology and Laboratory Medicine, Weill Cornell Medical College, 1300 York Avenue, New York, NY 10064, USA
Abstract
Organ regeneration promises unlimited access to replacement tissues. The current paradigm of organ regeneration requires transplantation of adult tissue-restricted stem and progenitor cells to repair the damaged organ. However, healing injured organs often leads to fibrosis with little recovery of function. An alternative approach is to harness the regenerative activity of microvascular endothelial cells (ECs) to support endogenous organ repair. Recent work demonstrates that organ regeneration can be directed by paracrine mediators, called “Angiocrine Factors,” elaborated by tissue-specific ECs to support stem and progenitor cells to directly induce organ regeneration without maladaptive fibrosis. Yet, the regenerative function and the repertoire of angiocrine factors elaborated by ECs depend upon the organ from which they originate.
New technologies have emerged to transcriptionally reprogram amniotic fluid cells (ACs) into generic “unspecified” ECs that acquire tissue-specific function promises a ready source of transplantable ECs to be used for organ regeneration. Generic AC-derived ECs can be induced to acquire organ-specific functions by a process of “in vivo education” wherein extravascular cues trigger transcriptional programs within engrafted ECs enabling them to acquire tissue-specific functions and to deploy angiocrine growth factors that drive organ repair without aberrant pro-fibrotic remodeling. Identifying tissue-specific transcription factors regulating tissue specification of EC is at the frontier of this new approach for organ regeneration.
The chapter is expected to overturn the scientific conceptualization of a monofunctional, inert, microvasculature by revealing a dynamic, tissue-specified role for ECs in organ repair that will enable therapeutic use of “educated,” tissue-specified ECs that home to their native injured organs and supply tissue-specific angiocrine signals to orchestrate organ regeneration.
1 Introduction
Organ regeneration promises unlimited access to replacement tissues to treat a wide variety of human diseases. However, clinical scale production of cells with regenerative function is difficult. Reprogramming human amniotic fluid cells (AFCs) offers a new and potentially better way to promote organ regeneration via cell-based therapies. Vascular endothelial cells (ECs) function not just as passive blood conduits but also provide regenerative cues to damaged tissues, but ECs are difficult to propagate in vitro from primary cell cultures. Advanced technologies allow, for the first time, efficient generation of ECs by “reprogramming” differentiated cells such as human AFCs. AFCs appear to have unique attributes that permits stable and efficient reprogramming and promise to make AFC-derived grafts available to promote regeneration and potentially to directly treat vascular diseases. AFCs can be directly reprogrammed into fully functional ECs without passing through a pluripotent state thereby avoiding the potential for teratoma formation. Derivation of patient-specific ECs derived from banked human AFCs is expected to facilitate development of customizable, functional grafts on-demand, matched to the immune profile of a recipient receiving a new class of personalized, reconstructive therapy for tissue damage resulting from disease or trauma. The selection of donor cell sources, derivation technologies, delivery approaches, and definition of the end points used to assess effectiveness are all areas of active investigation. Ultimately, success will require the availability of high-quality regenerative cells produced from well-defined sources on a clinically relevant scale with standardized functional characterization.
This chapter highlights recent advances in reprogramming assays of human AFCs that have lead to a new generation of fully viable, functional, three-dimensional endothelial cells. “Reprogramming” human AFCs to generate clinically scaled, functional ECs is now possible by enforced expression of vascular directing ETS genes. This new technology appears to more efficiently, reliably, and stably yield functional ECs thereby circumventing the limitations of strategies that rely upon directed differentiation of pluripotent stem cells.
1.1 Endothelial Cells and Amniotic Cells
Stretched out, the circulatory system of each person extends over 100,000 miles [1]. Thus, each human has enough endothelial cells (ECs) lining the inner surface of the circulatory system to wrap around the earth two to four times (equator: 24,901.5 miles). We think of the endothelium as the passive lining of blood and lymphatic vessels but recent studies clearly show that ECs also provide instructive cues to host tissues. ECs within each organ system are highly tuned to their resident tissues. As a result, ECs within the body are heterogeneic with each organ hosting unique tissue-specific (organotypic) ECs.
Regenerative cell therapy requires large numbers of engraftable cells. It has been difficult to produce enough progenitors in vitro despite the development of highly refined cell culture conditions and cytokines. Tremendous efforts have so far failed to capitalize on the promise of pluripotent stem cells (PSCs)—such as embryonic stem cells (ESCs) and induced pluripotent stem cells (iPSCs)—to produce cells with regenerative function. This approach is hampered by the low efficiency with which grafts can be produced and the general instability of the differentiated cell progeny. Adult stem and progenitor cells, with more restricted potential such as mesenchymal stem cells (MSCs), hematopoietic stem and progenitor cells (HSPCs), and endothelial progenitor cells (EPCs), can be useful if sufficient numbers of cells can be obtained. However, expansion of these cells in vitro typically leads to divergent differentiation yielding highly heterogeneous cell populations. A new approach is to instead, produce cells that can direct host-resident progenitors towards their regenerative potential. ECs have this capacity via production of instructive, angiocrine, factors that spur-resident tissue stem and progenitor cells to repair tissue damage. This approach promises that EC donor grafts could be used to promote organ regeneration. For this strategy to work, one needs access to a sufficient number engraftable ECs with organ-specific angiocrine function.
The precise definition of differentiated ECs remains a matter of debate. Nevertheless, to date ECs are widely defined as plastic- or extracellular matrix (ECM) proteins (collagen, fibronectin) [2, 3] adherent cells that, under closely controlled conditions, express EC-specific lineage markers (VE-cadherin, CD31, KDR, vWF). Cultured ECs can uptake acetylated LDL, bind to Ulex europaeus lectin, and respond to exogenous growth factors [4, 5] and environmental cues such as shear stress [6]. Both genotypic and phenotypic characteristics of differentiated endothelial-like cells fully depend upon formulation of the media composition that determines efficacy and functional potential. As yet, few if any endothelial-like cells produced from pluripotent or adult stem cells express all characteristics of genuine ECs relevant to human cell therapy.
Recently, “direct reprogramming” of terminal somatic cell from various tissues that trans-differentiate into linage committed ECs has emerged as an attractive new approach. Human dermal fibroblasts have been trans-differentiated towards ECs during in vitro culture on gelatin scaffolds in endothelial growth medium (EGM) [7, 8]. Direct reprogramming of functional vascular ECs from AFCs by forced ectopic expression of endothelial-specific transcription factors has also been shown to reliably yield naive ECs that acquire tissue-specific functions after transplantation into regenerating organs [9, 10].
Human AFCs are an ideal population of nonvascular cells amenable to reprogramming into authentic vascular ECs. Amniotic fluids are routinely cultured from the amniocentesis of mid-gestation human fetus for diagnostic purposes and have received significant attention for their potential to differentiate into various cell types [11, 12]. Now, standardized methods enable reliable derivation of functional vascular ECs that circumvent limitations of PSC-based approaches and are suitably scalable for the development of regenerative cellular therapy. Such engineered endothelial grafts for organ regeneration are also well suited to promote revascularization of the regenerating organ thereby restoring blood flow and maintaining the graft after implantation. Direct reprogramming of human AFCs using defined ETS genes to generate generic ECs offers the potential for high-throughput large-scale production of endothelial grafts for use in regenerative medicine applications.
1.2 Vascular Beds Instruct Organ Regeneration via Angiocrine Factors
Endothelium is a distinct inner layer of cells that form highly branched tubular vascular networks connecting tissues to their environment. ECs are well positioned to regulate respond to changes in the microenvironment by altering gene-encoded metabolic pathways. Capillary bed ECs have numerous surface projections to form the smallest vascular systems, called microvessels or microcirculation. Capillary walls are composed of single layer of EC closely associated with pericytes via microvilli and filopodia. Sinusoidal ECs are a discontinuous endothelium anastomosed to capillary beds that can acquire organ specification in response to tissue-specific microenvironment-derived signals that influence the morphogenesis of endothelial heterogeneity.
The ECs in direct contact with blood are called vascular endothelial cells and form arteries and veins, whereas ECs are in contact with lymph fluid are knows as lymphatic EC. Vascular and lymphatic ECs line the entire circulatory system in the body. The lymphatic ECs allow the lymphatic vasculature to drain interstitial fluid. Vascular and lymphatic ECs are distinguished by their distinct expression of molecular signatures that define and likely contribute to their functional and morphological differences. Understanding the physiological role of ECs has been mainly directed by studies of their role in tissue homeostasis as vascular conduits, that is their role in blood and lymphatic plumbing. Yet the lining of these structural conduits have a myriad of functional roles in blood circulation: modulating blood coagulation and fibrinolysis; setting vascular tone; mediating blood–tissue exchange and fluid filtration; responding to biomechanical factors such as shear and tensile stress; altering the metabolome and hormone trafficking; and as gatekeepers selecting or refusing migration of blood and bone marrow (BM) cells.
More recently, ECs have also been shown to support and direct adult stem and progenitor cells during homeostasis and organ regeneration by elaborating juxtacrine/paracrine molecules referred to as “angiocrine factors.” ECs with such function comprise an “endothelial instructive niche” that orchestrates organ regeneration/repair [13]. Angiocrine factors central to niche function include juxtacrine signaling via the Notch pathway (Notch-1, Notch-2) and cytokines with trophic paracrine activity on neighboring cells [14]. Angiocrine factors provide local instructive cues within damaged tissues to direct regeneration mediated by resident stem and progenitor cells. ECs deploy distinct angiocrine factors in response to particular microenvironmental cues and these adaptive functions provide fine control over stem cell proliferation and differentiation. In pathologic conditions, tumor cells appear capable of co-opting ECs to provide pro-tumorigenic angiocrine/niche functions [15].
The processes of repair, remodeling, and regeneration generally restores tissue continuity by replacement of lost cells and synthesis of the ECM proteins that bind them. Specialized cells are recruited to the site of regeneration, and undergo differentiation, replication, re-specialization, and go on to replace the missing tissue. Host tissue stem cells are induced to proliferate and differentiate as necessary to regenerate the lost tissue. An instructive role of sinusoidal ECs in organ regeneration was shown in mouse liver and lung resulting from the interplay of cytokines, growth factors, and metabolic pathways at the injury site of injury. During regeneration, cell traffic dynamics are altered at the original anatomical site via modulated expression of chemokines expressed by ECs to augment recovery of the missing organ mass. For example, liver sinusoidal ECs—immunophenotypically defined as VEGFR3+/VEGFR2+/VE-cadherin+/FactorVIII+/CD34−/CD45− cells—releases angiocrine factors that initiate and sustain liver regeneration induced by 70 % partial hepatectomy [16]. Priming of the vascular niche by organ injury prepares the way for new tissue formation by providing a vascular network employing essential instructive cues at the anastomosed sites to promote repair and regeneration of the damaged tissue. These regenerative functions of ECs are normally dormant, adaptively revealed during recovery from tissue damage. The nature and extent of endothelial plasticity is poorly understood and its centrality to regeneration is only starting to be appreciated.
1.3 Endothelial Cell Plasticity, Ontogeny, and Reprogramming
Much has been learned about cell fate plasticity from recent efforts to directly reprogram terminally differentiated somatic cells to other cell types. Such transdifferentiation captures nascent cellular plasticity—typically by enforced expression of lineage-specifying transcription factors—to drive epigenomic remodeling towards other potential cell fates. Normally, “plasticity” permits a cell to cope with environmental/conditional variations. A clear example of reprogramming occurs within the zygote following fertilization. In vitro, somatic cell nuclear transfer (SCNT) enables the reprogramming factors in the ooplasm of an enucleated egg to faithfully reprogram the epigenome of a donor nucleus to other cell types, so called “nuclear reprogramming” [17–19]. In addition, differentiated cell types can be reprogrammed by treatment with pluripotent cell extracts [20–22]. The largest impact has resulted from the observation that enforced expression of a small number of transcription factors can reprogram terminally differentiated cells to iPSCs [23].
It is now recognized that not all methods of reprogramming are suitable for all cell types. A major determinant of cellular plasticity is the cell’s intrinsic epigenetic state that defines its ability to switch cell fate. DNA methylation plays a significant role in reprogramming because agents that demethylate DNA methylation can enhance the efficiency and effectiveness of cellular reprogramming [24]. It is clear that reprogramming requires both a permissive epigenetic “ground state” amenable to complete reprogramming and the correct “cocktail” of reprogramming factors to direct the transition.
Some cell types are normally plastic. Plasticity is a predominant feature of adult stem cell types permitting them to differentiate towards multiple lineages.
Plasticity of endothelial progenitors was first observed during embryonic development in primordial ECs and hematopoietic cells of the yolk sac [25–27]. Emerging from these studies is the concept of a hemangioblast, the common mesodermal precursor of vascular and hematopoietic cells [28]. This ontogeny persists in the definitive embryo and critical fate decisions rely upon the differential expression of particular transcription factors. Hemogenic endothelium—endothelial precursors that can yield either definitive endothelium or hematopoietic cells—is transiently generated and appears dependent upon expression of Scl/Tal1 and perhaps ETV2 [29–31]. The transcription factor Runx1 is then required to generate definitive hematopoietic cells from hemogenic endothelium [32], suggesting this master regulator directs endothelial precursors to hematopoietic fates. The central role of epigenetic regulation is demonstrated by the inappropriate expression of neural genes in hemangioblasts when components of the polycomb repressive complexes 1 (PRC1) were deleted [33]. Thus, hemogenic endothelium must be epigenetically poised for both endothelial and hematopoietic futures but not other possible fates.
The hierarchy of vascular identity is initiated early during embryogenesis. Endothelial progenitors are modeled towards arterial or venous fates before endothelial tubes form in the chick embryo [25, 26]. Yet embryonic ECs also retain arterial–venous plasticity until late in embryonic development [13, 34]. Adult ECs also retain remarkable plasticity and a large body of work suggests that plastic, EPCs persist throughout life. It is perhaps from such endothelial progenitors that the tissue-specific characteristics of microvascular ECs emerge after being engrafted into host tissues [35]. Indeed, heterogeneity of the angiocrine signals deployed by ECs in different tissues is only now emerging [14, 16, 36, 37]. This heterogeneity means that regenerative therapies attempting to replicate the angiocrine function of organ-resident endothelium must recapitulate the organ-specific angiocrine regenerative functions provided by endothelial grafts.
One approach is to generate large numbers of naive endothelium that engraft damaged tissues where they acquire the necessary angiocrine functions to promote regeneration. That EPCs can only be purified in small numbers is a significant hurdle to their clinical application. So other approaches are being taken. PSCs can be directed to endothelial fates but current methods yield only small numbers of cells with endothelial features (Daylon papers). An ongoing challenge of grafts generated by in vitro directed differentiation of pluripotent cells is residual plasticity that allows the progeny to drift to other fates or yield teratomas. It is now clear that differentiation from a pluripotent state is unnecessary and perhaps detrimental.
Mature somatic cells harbor residual plasticity that can be captured by the enforced expression of transcription factors that induce their conversion to other cell types. Such cellular interconversions elicited experimentally often mimic aspects of developmental cell fate transitions and it is possible that the two processes are fundamentally similar. As early as 1987, MyoD1 was shown to convert fibroblast to skeletal myocytes [38, 39]. Additional examples of such direct reprogramming of mature somatic cells provide important insights into the core transcriptional networks and cellular signaling pathways required for intra- and inter-lineage fate transitions [40, 41]. It remains an open question whether direct reprogramming of mature cells will circumvent the challenges identified in regenerative grafts derived by directed differentiation of pluripotent cells.
2 Transcriptional Regulation of Stage-Specific Endothelial Differentiation: Mini Review
A dynamic morphogenic process that all cells come from pre-existing cells is the mean to measure “different” cell identity, and the morphological adaptation steps in relation to cellular function are called “differentiation.” Differentiation is determined by a cell fate and by spatiotemporal specification. A cell fate is identified either by autonomously (committed), conditionally (reversible), or syncytially “specified” or by “determined (irreversible)” to corresponding progeny,1 and relies on cellular morphogenesis property. Major advances in molecular tools and microscopy technology have been transformational in understanding the mechanisms that drive the specification for a cell fate by tracing cell lineages during differentiation steps. Cell lineage tracing (or “fate mapping”) provide information about a differentiation to learn about the actual changes in biochemical and structural status, functional morphometric characterization, biointerface communications, and phenotypic adaptations in an incessant signaling feedback that governs cellular fate.
Experimental findings on in vitro directed differentiation of stem cells suggests that PSCs can unlimitedly differentiate into ECs. Embryonic stem (ES) and iPSCs represent PSCs, and have been an in vitro models to analyze molecular mechanisms underlying differentiation steps to generate ECs through sequential differentiation steps through embryoid body (EB) formation that mimics the in vivo vascular morphogenic process, followed functional development into arterial, venous, capillary, and lymphatic ECs, as well as smooth muscle cells [42]. In vitro culture condition for PSCs can be highly defined, xeno free and/or serum free for accurate profiling in molecular pathways governing development of ECs and assembly of complex vascular networks occur in vasculogenesis, angiogenesis, lymphangiogenesis, and vascular remodeling. Nonetheless, the PSC culture platform can identify populations of reprogrammed ECs derived from various cells including PSCs, mesenchymal stroma cells (MSCs), and AFCs from amniotic tissues, as well as to a variation of signaling pathway attribute governing the maintenance of different PSC types, including leukaemia inhibitory factor (LIF) and bone morphogenetic protein (BMP) for mouse ESCs, a nodal factors, activin/inhibin/transforming growth factor beta (TGFβ), and fibroblast growth factor (FGF) for human embryonic stem cells (hESCs).
Recent advances in direct reprogramming of somatic cells to stem cells or to other type of somatic cells via epigenetic reprogramming by forced expression of specific transcription factors of a different cell fate enriched in the target cell suggest that the erection of a cell fate switch could be a key event in induced differentiation. This approach has been successful in generating functional ECs. The process in general requires an epigenetic reprogramming achieved by the transcription factors that can directly interact to chromatin for epigenetic modification, followed by exposure to specific developmental cues to generate cell populations of a given fate. This forced lineage conversion has attracted increasing attention as a potential alternative to the directed differentiation of PSCs to obtain cells of a given lineage. Importantly, although a biochemistry description of cellular genetics and epigenetics is taken significant credit for differentiation, additional players have recently reported, which may provide better understanding for the differentiation which one day will lead to more facile and efficient manipulation of cell fate for regenerative medicine. As such, specific noncoding RNAs and micro RNAs can be used to mark to track and predict for cell fate switch that can be used to directly manipulate cell fates.
The question remains as to which development stage represents the best candidate for the strategy to generate such ECs with the best functional benefits which account with enhanced angiogenesis, reduced vascular remodeling, or to cytokine-mediated effects that promote the survival of endogenous cells. Pluripotency-associated factors and lineage specifiers have generally been considered to determine the identities of pluripotent and differentiated cells, respectively [43, 44]. The pleiotropic expression patterns of embryonic genes that are usually suppressed in definitive developmental stages, and are persistently present in the PSC cultures in developmental differentiation process is governed by dynamic interchangeable regulation of heterogeneity. A hallmark of PSCs, contributing to plasticity to lineage specification to EC is shown driven by reduction of the transcribed portion of the genome [45]. Different stages of developmental differentiation may be determined by the different set of signals by the targeted activation of stage-specific genes or of lineage-specific genes or by selective silencing of genome regions. Stage-specific transcriptional expression of the targeted cells may sporadically enhance or suppress tissue-specific genes, is called cell plasticity that is accompanied by disproportionate expression of set of chromatin-remodeling genes and the transcription machinery. This transcriptional hyperactivity landscape in the target cells can be profiled using whole-genome tiling arrays, i.e., high-throughput RNA sequencing.
2.1 Endothelial Cell Identity by Transcriptional Regulation
Understanding the cellular identity that can be grafted to give rise to large number of functional ECs without developing malignant outputs is a major goal of directed reprogramming strategies for clinical therapy. During early commitment to a mesodermal lineage, the complex transcriptional and epigenetic changes are associated to EC lineage commitment process, and tens of transcription factors have been identified. ETS family factors specify cell autonomous function of de novo vasculogenesis and myelogenesis by direct interaction with promoters that can switch on vascular-specific genes by efficient transcription of signaling in angiogenic repertoire evident in adult vasculature. ETS binds to endothelial promoter/enhancer and directly regulates expression of VE-cadherin [46, 47], Tie/Tek [48], Flk-1 [49, 50].
In the developing endothelium, the ETS proteins function redundantly with the exception of non-redundant role of Etv2/ER71 as a primary player in specification and differentiation of the endothelial lineage [51]. The endothelial-specific zebrafish Ets1-related protein (Etsrp) is functional orthologs of human Etv2/ER71 (zebrafish: Etsrp, mouse: ER71, human: Etv2/ER71) that assume regulation of endothelial gene expressions. Etsrp, which expression is restricted to endothelial precursors and differentiated ECs in the zebrafish embryos [52], has been identified as a primary player in specification and differentiation for vascular reprogramming that activates other ETS transcription factors. Overexpression of mouse ER71 and zebrafish Etsrp caused strong expansion of hemangioblast and vascular endothelial lineages [53]. Analysis of one of ETS family of transcription factor, fli-1 in the zebrafish embryo has identified that the initial expression of fli-1 in the posterior lateral mesoderm is the earliest indicators of hemangioblast formation [54].
Using microarray profiling and genetic tracking for the directed differentiation of hESC into embryonic ECs, ER71, Fli1, and Erg isoform 2 (Erg-2) have been reported as key ETS transcription factors that are expressed during differentiation of hESCs into ECs [10]. More recently, high-throughput RNA-sequence analysis revealed that adult vascular ECs constitutively express Etv2/Er71, Fli1, Erg, Ets1, Ets2, Elf1, Elk1, Vezf, and Etv6, while Etv2/ER71 is only transiently expressed during the early phases of fetal development specifying vasculogenic differentiation of hESC-derived mesodermal precursor cells, and its expression is shut off in adult vascular ECs [9]. Whereas, other ETS transcription factors expressed in committed ECs, such as Fli1, VEZF, and ETV6, as well as various Erg and Elf isoforms are constitutively expressed during fetal development and adult ECs.
In addition to the ETS family, several transcription factors are known to induce endothelial-specific gene expression. In adult EPC precursor cells, the endothelial nitric oxide synthase (eNOS) promoter was shown silenced by DNA methylation and prominent repressive histone H3K27me3 marks, and silencing of histone demethylase, Jmjd3, indicating that hypoxia plays a role in recruiting EPC precursor cells in ischemic tissue that facilitates eNOS expression via activation of Jmjd3 that can turn on the epigenetic modifications [55]. An involvement of FBW7 in regulation of endothelial function in migration, angiogenesis, inflammation, and barrier integrity has been shown that FBW7 targets the zinc finger transcription factor Krüppel-like factor 2 (KLF2) in ECs upon activation of KLF2 by glycogen synthase kinase-3 (GSK3) at two conserved phosphodegrons [56, 57].
Implications for gene regulation during development are more complex involved in the case for co-activator and it’s binding to enhancer of the activating genes. In hemogenic endothelium of mouse ESC, a hemogenic endothelial development regulator, Sox17 has been known expressed. Sox17 is suggested that it plays a pivotal role in the development and/or expansion of hemogenic endothelium through the Notch signaling pathway [58]. During the differentiation of hESC to EC, forkhead box protein A2 (FOXA2), also known as hepatocyte nuclear factor 3-beta (HNF-3B), or as transcription factor 3B (TCF-3B), has been identified differentially expressed transcription factor specific to early mesoderm commitment, which is bivalently marked with histone modifications for both gene activation (H3K4me3) and repression (H3K27me3) in early stage of differentiation of hESCs to mesoderm [59]. Cooperation between KLF2 with ERG was suggested that synergistically activate transcription of Flk1 while KLF2-binding site is located on the Flk1 enhancer region [60]. The mitogen-activated protein kinase (MAPK) family of transcription factor, Foxo1 (FKHR) has been shown to interact with Ets-1 and functions as a coactivator for Ets-1 on Flk-1 promoter in bovine carotid artery ECs [50]. Myocyte Enhancer Factor 2 (MEF2) family of transcription factors that is expressed very early in the development of the endothelium, has been identified for the enhancer gene with four consensus Ets transcription factor-binding sites that is upstream of MEF2-binding site [61]. Zebrafish hemangioblast gene Cloche was identified to act upstream of the homeobox gene, Hhex (Flk-1 homologue), in blood island [62]. A zebrafish homolog of Etv2/ER71, Estrp, was identified as an upstream regulator for Cloche and SCL in the pathway from mesodermal precursor to hemangioblast. F-box and WD repeat domain-containing 7 (FBW7), the substrate-binding subunit of E3 ubiquitin ligase SCF (FBW7) (a complex of SKP1, cullin-1 and FBW7) is required for vascular development.
The lymphatic vasculature forms during embryogenesis through vasculogenesis via predominant means of vessel formation after arterial–venous EC specification within hemangioblast mass [63] via transcription regulation switch into a thin-walled, blind-ended lymphatic vessels with valves to transport fluid to the lymph node comprised a single, non-fenestrated EC layer [64]. The lymphatic network forms a second vascular system lined with vascular ECs, which complements the vascular network by modulating tissue fluid balance, allowing interstitial protein transport, and initiating the immune response [65].
VEGF-C/VEGFR-3 signaling is well known for lymphatic EC sprouting after lymphatic EC specification by Prox1 that can rescue lymphatic EC sprouting in whole-mount explants of this mutant LYVE-1 and podoplanin [66]. The sequence, organization, and variation of lymphatic ECs are regulated by the VEGFR3 gene which proximal promoter is TATA-less that contains stretches of sequences homologous with the mouse Vegfr3 promoter region, and control EC-specific transcription of downstream reporter genes, suggesting that EC-specific elements occur in the proximal promoter, although further enhancer elements are probably located elsewhere [67]. Several transcriptional factors regulate VEGFR3 expression have been reported, Spred/Sprouty family proteins, negative regulator for growth factor-induced ERK activation, Spred-1/2 has been shown involved in lymphangiogenesis by negatively regulating VEGF-C/VEGFR-3 signaling [68]. Induction of VEGFR-3 expression by VEGFR-3 promoter binding is also possible by the Notch-1 complexed with DNA-binding protein CBF-1/suppressor of hairless/Lag1 (CSL) [69].
More recently, mutation in transcription factor coding gene sex determining region Y [SRY]-box 18 (Sox18) was shown selectively impaired lymphatic sprouting from the cardinal vein and resulted in defective lymphatic thoracic duct formation, suggesting that a cross talk between the growth factor and transcription factor pathways regulate lymphangiogenesis [70]. In mouse dorsolateral sector of the cardinal vein at E9, a subpopulation of venous ECs has been identified to acquire to express the transcription factors, Sox18 approximately 1.5 day prior to the onset of prospero-related homeobox 1, a homeobox transcription factor (Prox1) and podoplanin [71]. In human, COUP transcription factor 2 (COUP-TFII), also known as nuclear receptor subfamily 2, group F, member 2 (NR2F2) has been shown required to activate Prox1 through direct binding to the Prox1 promoter region [72], suggesting that an acquisition of Sox18/COUP-TFII is essential for venous ECs to switch into lymphatic fate, and upregulation in Prox1 is associated with induction of lymphangiogenesis. In mouse ESC-derived Sox18+ lymphatic vessels, forced expression of Prox1 induces suppression of Sox18 expression, and concomitant upregulation of FoxC2, angiopoietin-2 and HoxD8. Furthermore, HoxD8 was found upregulate Prox1 expression, indicating a positive-feedback-loop mechanism in which Prox1 expression specified by HoxD8 [73]. This suggests that Sox18-Prox1-HoxD8 axis may play a pivotal role in the regulation of lymphangiogenesis. It is, however, not clear yet what molecular mechanisms are responsible for the restriction of its expression to the lymphatic endothelium during development.
2.2 Genetic Regulator and TGFβ for Endothelial Function
The TGFβ signal inhibition has been shown essential for a reprogramming of human AFCs into EC with transient expression of Etv2/ER71 and constitutive Fli1 expression [9, 74]. The vascular growth requires a bifunctional regulator, TGFβ signals that are activated by contact between EC and mesenchymal cell, modulates vascular growth [75, 76] by a punctuated pattern of TGFβ expression that is set aside a ventral mesodermal population. As such, low dose upregulate angiocrine factors, whereas high doses appear to inhibit EC growth [76–78], which low dose expression is required in the de novo synthesis of primordial vasculature. As such, suppression of nonvascular genes attenuated by TGFβ signal inhibition is essential for the assembly of VEGFR2 signaling pathway and remodeling, which results in VEGF-A-induced expansion of large numbers of reprogrammed ECs via interaction with pericytes or vascular smooth muscle cells [74].
TGFβ has been known its bifunctional role for vascular cell growth and differentiation in vascular formation via induction of mural cell development, activation and inhibition of EC proliferation and differentiation, augmenting EC migration via alterations in EC matrix deposition [79–81]. TGFβ-deficient mice die in mid-gestation from defective yolk sac vasculogenesis ranging from delayed vasculogenesis to the total absence of vessels, thus implicated important in hereditary hemorrhagic telangiectasia (HHT), atherosclerosis, tumorigenesis, and immunomodulation [82–84]. The TGFβ family members, TGFβ1, TGFβ2, TGFβ3, BMPs, activins, inhibins, and endoglin, bind to two types of a serine threonine kinase receptors, type I (Alk1, Alk5), type II (TGFβRII), and type III (Endoglin) that are also expressed on ECs [85]. Although TGFβ signaling is not required for differentiation of extraembryonic mesoderm into ECs, it is necessary for their subsequent organization into robust vessels. A major function of TGFβ in yolk sac mesoderm is to regulate production and deposition of fibronectin in the ECM that maintains yolk sac integrity, which TGFβ signals are tightly regulated for the differentiation of hemangioblasts [86]. With targeted deletion of TGFβ1, half of the mutant embryos die at E9.5-E10.5 due to defective yolk sac vasculogenesis while half survive several weeks before succumbing to inflammation [83, 86, 87]. Deletion of TGFβRII [88, 89] as well as ablation of Alk1 or Alk5 [84] causes in embryonic lethality due to defective vasculogenesis in the yolk sac, suggesting that these TGFβ signaling pathways are not redundant. Alk1 phosphorylation activates smad1, smad5, and smad8 whereas Alk5 activates smad2 and smad3, which then complex with smad4 to activate transcription in the nucleus.
TGFβ induces a number of genes associated with the ECM on ECs, including fibronectin and collagens I, IV, and V [85]. Mice lacking one of TGFβ family, endoglin, also known as CD105, END, FLJ41744, HHT1, ORW, and ORW1, die from defective vascular development by gestational day 11.5 caused poor vascular smooth muscle development [90–92], by arresting endothelial remodeling with vascular malformations [83, 93]. ETS factors regulate matrix turnover by activating transcription of several metalloproteinases (MMPs), that was shown in the study that Ets1 undergo acetylation in response to TGFβ signaling [94]. TGFβ was shown to affect the transactivation activity of Ets-1. TGFβ augments the transactivation activity of Ets-1 by inducing a protein that interferes with the binding of Ets-1 to the DNA-binding site. This study also showed that Ets-1 expression to the level equivalent to FGF2 enhances DNA-Ets complex formation, whereas TGFβ attenuates basal and FGF2-enhanced DNA-Ets complex formation via the promoter activity driven by Ets-1 [95].
A beneficial effect of TGFβ inhibition attenuated on the expansion and preservation of vascular phenotype in ECs derived from hESC that are differentiated to embryoid body (EB) culture on matrigel-coated plates in serum-free media supplemented with VEGF-A [10]. The proliferation of vascular cells resulting in a net 36-fold expansion into EC-like cells exhibited a transcriptional profile of Id1(high)VEGFR2(high)VE-cadherin(+) ephrinB2(+) that Id1 is required for increased proliferation and preservation of EC lineage commitment [10]. The contribution of TGFβ signal inhibition to the ETS transcription factor mediated reprogramming into ECs was also shown a significant decrease in total VEGFR2 expression, which confirmed that TGFβ signaling regulates VEGFR2 expression in EBs [96]. More recently, inhibition of TGFβ was shown essential for transcriptional direct reprogramming into function ECs with forced expression of transient Etv2/ER71 with concomitant Fli1/Erg1 derived from human amniotic fluid-derived cells (AFCs) of second trimester amniocentesis. These data suggest that in combination with overexpression of ER71 and Fli1, complete reprogramming into ECs requires TGFβ inhibition to induce and maintain the expression of functional VEGFR2 signaling apparatus [9].
2.3 The Production and Directed Differentiation of Embryonic Stem Cells into Endothelial Cells
The prospects for use of hESCs derivatives in regenerative medicine are significant, and there is much optimism for their potential contributions to human regenerative medicine. The methods for deriving hESCs are well established from chromosomally euploid, aneuploid, and mutant human embryos that are available from in vitro fertilization (IVF) clinics treating patients for infertility or preimplantation genetic diagnosis (PGD) from four- to eight-cell morula and from blastocysts and from isolated inner cell mass (ICM) cell clusters. The ESCs can be formed and maintained on human somatic cells in xeno-free, serum-free culture system that can spontaneously differentiate when grown in the absence of the feeder cells, when overgrown in culture, or when removed from the maintenance colony. All three major embryonic lineages are produced in differentiating flat attachment cultures and unattached embryoid bodies (EBs). Stage-specific differentiation can be identified by stage-specific surface markers, or by expression of reporter genes, as well as by characteristic morphology. Directed differentiation methods are well documented for ectodermal lineage (neural cells) and mesendodermal lineage (cardiomyocytes, hematopoietic cells, endothelial cells), but few for endodermal lineage, which may be due to limited arrays of stage-specific markers available for endodermal lineage [97].
A derivation of mouse and rat ESCs from the late epiblast layer of post-implantation embryos and long-term maintenance demonstrated using chemically defined, activin-containing culture medium that a direct differentiation of ESCs into specific adult cell types is regulated by endoderm organogenesis via a nodal signal, activin/TGFβ, that promotes the expression of a conserved transcription factor network; a mix-like homeobox genes Foxa2, Sox17, Eomesodermin, and Gata4-6 [98]. This network of transcription factors can activate a cascade of gene expression that functions to commitment to an endodermal fate, segregate the endoderm and mesoderm lineages, and integrate signaling events that regionalize the nascent endoderm [99, 100], suggesting an evolutionarily conserved role in the derivation and the maintenance of pluripotency in stem cell. The precise roles of these factors vary between species, thus distinguishing human progenitors requires purification schemes based on additional information.
Reported in 2002, the function of BMP4 was identified that BMP4-induced emergence of trophoblasts from hESCs [99, 101]. Conversely, BMP4-treated hESCs may endogenously expressing trophoblast gene via induction of mesoderm by cooperating with FGF2 that act through BRA and CDX2 [102]. Similarly, directed hES differentiation into the definitive endoderm lineage in the presence of a nodal signal, activin A, and low serum culture condition was shown possible for up to 80 % definitive endoderm cells, which both early endoderm and mesoderm genes were co-detected when enriched using CXCR4 [103], which was confirmed in visceral endoderm of mouse embryos [104]. Mouse ESCs emerge in response to 3-day treatments with BMP4 or retinoic acid that promotes development of posterior epiblast precursors, which give rise to primitive streak mesoderm, extra embryonic mesoderm tissues and primordial germ cells [105, 106]. Whereas retinoic acid has been shown to involve in the emergence of precursors that give rise to neuroectoderm and neural crest [107]. This selective culture condition deriving mixed meso-endoderm lineages using markers to monitor the differentiation using CD25, goosecoid (Gsc)+/Sox17+ definitive endoderm, and Gsc−/Sox17+ visceral endoderm, followed by the gene expression transcriptional profiling analysis of developmental genes [108]. BMP4-induced subsets of ESC culture was also shown to exhibit gene signatures of mesoderm, trophoblast, and vascular endothelium, suggesting correspondence to gastrulation-stage primitive streak, chorion and allantois precursors, respectively [104]. Same group identified that intermediate subset of cells positive to a receptor-related 2 (ROR2) produce mesoderm progeny, cells positive to aminopeptidase-A (APA) generate syncytiotrophoblasts, and cells positive to urokinase receptor (CD87) give rise to vasculature. ROR2, also known as tyrosine-protein kinase transmembrane receptor, or neurotrophic tyrosine kinase, is responsible for aspects of bone and cartilage growth. CD87, a GPI-linked receptor for urokinase plasminogen activator (uPA), is involved in the conversion of plasminogen to plasmin on the leading edge of migrating cells. Of all studies reviewing with a focus on early stages of endoderm formation how developmental mechanisms regulate endoderm organogenesis using PSCs, has emergence to a concept, epithelial-to-mesenchymal transition (EMT), in attention to a dynamic gene expression profile may be reminiscent of vertebrate gastrulation. However, whether endoderm cells emerge in whole or in part from mesendoderm progenitors have never been shown.
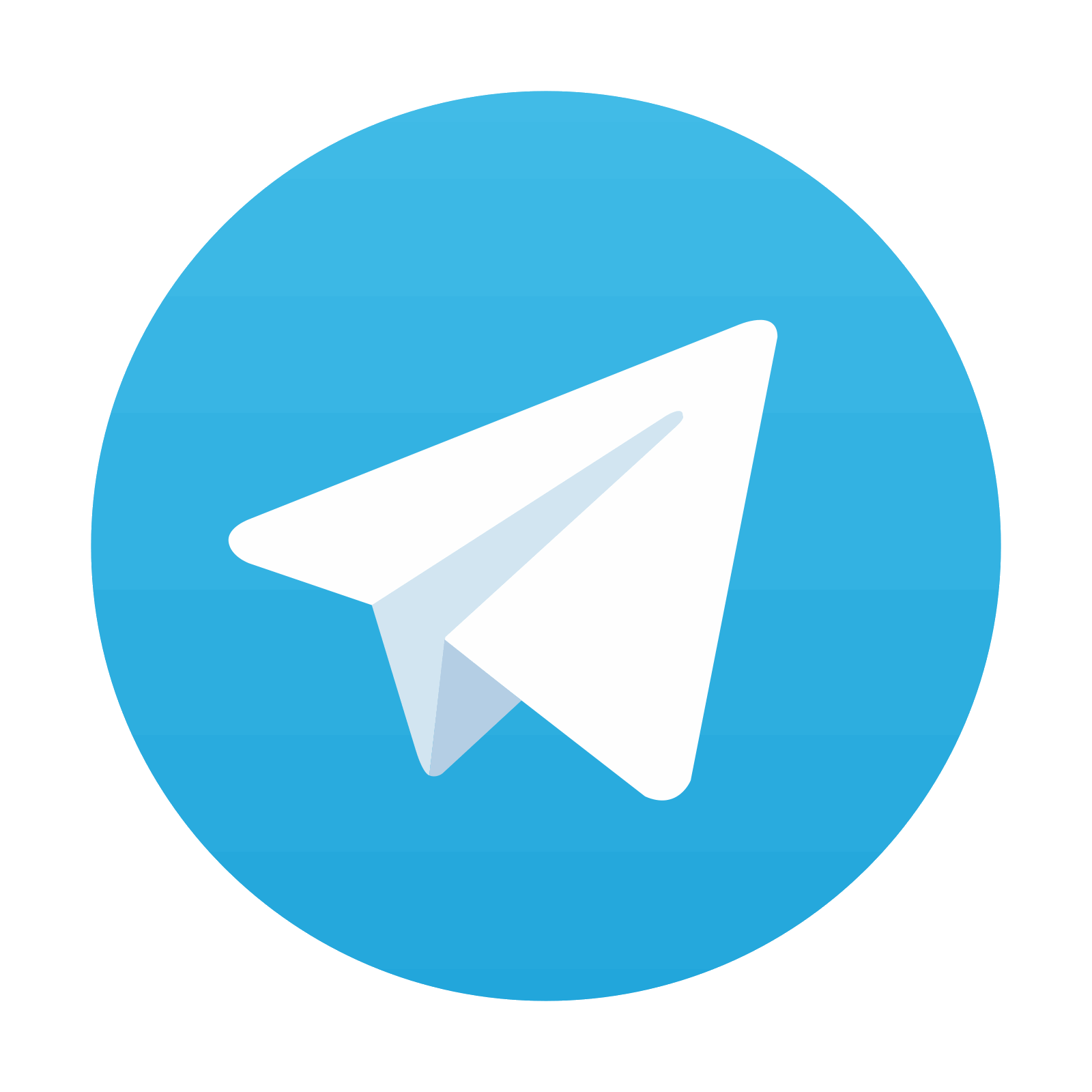
Stay updated, free articles. Join our Telegram channel
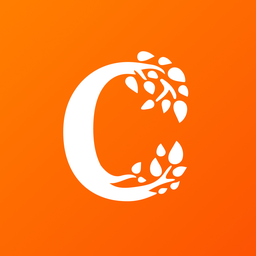
Full access? Get Clinical Tree
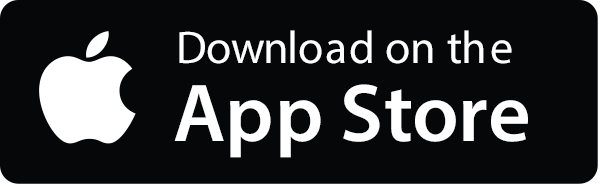
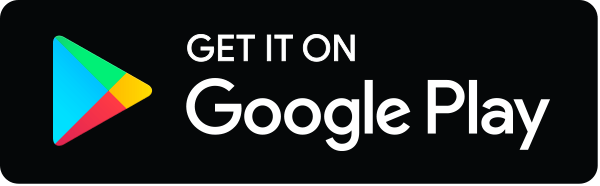