Fig. 2.1
Potential origins of genetic and epigenetic changes in the germ line. The “aberrant repair hypothesis” essentially posits that a variety of clinical, biological, lifestyle, and environmental factors including paternal age, obesity, gamete cryopreservation, exposure to radiation, transition metals, or a wide range of small molecular mass xenobiotics can trigger mitochondrial ROS generation and oxidative DNA damage in spermatozoa. These cells then fertilize the oocyte, possibly with the help of ART techniques such as ICSI (intracytoplasmic sperm injection), and it is then up to the oocyte to affect repair of the damaged paternal genome. Any inefficiencies or mistakes resulting from this round of postfertilization DNA repair have the potential to create mutations or epimutations that will impact upon the normal development and health trajectory of the offspring (Aitken and Krausz 2001)
Since DNA damage is such a common feature of defective spermatozoa generated by male-factor patients in an assisted reproductive technology (ART) setting (Lopes et al. 1998), it is inevitable that a significant number of in vitro conceptions are achieved using DNA-damaged spermatozoa that would have been excluded from this process in vivo. Although the use of ART and particularly ICSI (intracytoplasmic sperm injection) to achieve fertilization in cases of severe male infertility has been extremely successful in improving conception rates, such apparent therapeutic advances might have been achieved at a cost, in terms of the health and well-being of the offspring. Of critical importance to this discussion is a clear understanding of the oocyte’s competence for DNA repair. Typically, SSB are repaired by the base excision repair (BER) and nucleotide excision repair (NER) pathways, while DSB are repaired by nonhomologous end join (NHEJ) and homologous recombination (HR). The significance of these pathways in the oocyte has not been clearly articulated, but for the reasons given below, the BER pathway is probably the single most important DNA repair strategy associated with the oocyte’s central task of tackling the DNA damage brought into the zygote by the fertilizing spermatozoon.
It is the purpose of this chapter to examine the source and nature of DNA damage in human spermatozoa, to examine how such damage is repaired following fertilization, and to review how errors in this repair process impact on the health and well-being of the offspring. This discussion will begin with a consideration of the cause of DNA damage in human spermatozoa, and in this context, the spermatozoon’s susceptibility in oxidative stress is fundamental (Aitken and Clarkson 1987).
2.2 Oxidative Stress and Spermatozoa
2.2.1 The Perpetual Cycle of ROS Generation in Spermatozoa
A major cause of DNA damage in spermatozoa is oxidative stress mediated by a variety of reactive oxygen species (ROS) including free radicals such as superoxide anion (O2 −•), nitric oxide (NO•), or the hydroxyl radical (OH•) as well as powerful oxidants such as hydrogen peroxide (H2O2) or peroxynitrite (ONOO_). Spermatozoa are particularly prone to oxidative stress because their antioxidant defensive capacity is limited, due to the removal of a majority of their cytoplasm during spermatogenesis and a consequential reduction in cytoplasmic antioxidants such as catalase or superoxide dismutase. Furthermore, these cells are professional generators of ROS, with a vast majority of these reactive oxygen metabolites being generated as a consequence of electron leakage from the sperm mitochondria (Koppers et al. 2008).
The vulnerability of spermatozoa to oxidative stress also reflects the abundance of substrates these cells offer up for free radical attack. Thus, the membranous constituents of spermatozoa contain high concentrations of polyunsaturated fatty acids, particularly docosahexaenoic acid, the double bonds of which are vulnerable to attack by ROS and the initiation of lipid peroxidation cascades. The hydrogen abstraction event that initiates lipid peroxidation is promoted because the carbon–hydrogen dissociation energies are lowest at the bis-allylic methylene position, generating carbon-centered lipid radicals that then combine with oxygen to generate peroxyl (ROO•) and alkoxyl (RO•) radicals that, in order to stabilize, abstract hydrogen atoms from adjacent carbons. These chemical reactions create additional lipid radicals that then perpetuate the lipid peroxidation chain reaction, culminating in the generation of small molecular mass electrophilic lipid aldehydes such as 4-hydroxynonenal (4HNE), acrolein, and malondialdehyde.
Further to this lipid-based vulnerability to free radical attack, we have also recently demonstrated that the lipid aldehydes generated as a result of lipid peroxidation actually trigger the generation of yet more free radicals from the sperm mitochondria in a self-perpetuating cycle (Fig. 2.2). According to this scheme, lipid aldehydes such as acrolein or 4HNE form adducts with proteins in the mitochondrial electron transport chain (ECT) that perturb the normal, controlled 4-electron reduction of oxygen to water. This results in the leakage of electrons from the ECT that affect the 1-electron reduction of nature’s universal electron acceptor, oxygen, to generate O2 −•, which then rapidly dismutates to H2O2 under the influence of mitochondrial superoxide dismutase (Aitken et al. 2013). Intriguingly, defective spermatozoa from subfertile males contain a superabundance of superoxide dismutase, possibly reflecting the retention of excess residual cytoplasm during the terminal stages of spermiogenesis (Aitken et al. 1996; Gomez et al. 1996; Sanocka et al. 1997). The fact that correlations have also been observed between superoxide dismutase and other cytoplasmic enzymes such as glucose-6-phophate dehydrogenase or creatine kinase supports this view (Aitken et al. 1996; Gomez et al. 1996). Normally the presence of excess superoxide dismutase would be considered an asset for any cell seeking to protect itself from oxidative stress. However, unless this enzyme is accompanied by a corresponding increase in the presence of enzymes that can scavenge H2O2 such as glutathione peroxidase or catalase (both of which are present in limited supply in human spermatozoa; Storey et al. 1998; Sanocka et al. 1997), the presence of excess superoxide dismutase simply turns a short-lived, membrane-impermeant, relatively inert free radical in the form of O2 −• into a long-lived, membrane-permeant reactive oxidant, H2O2, that can attack a wide variety of substrates in spermatozoa. Such attacks not only target the polyunsaturated fats that abound in the sperm plasma membrane but also the DNA in the sperm nucleus and mitochondria.
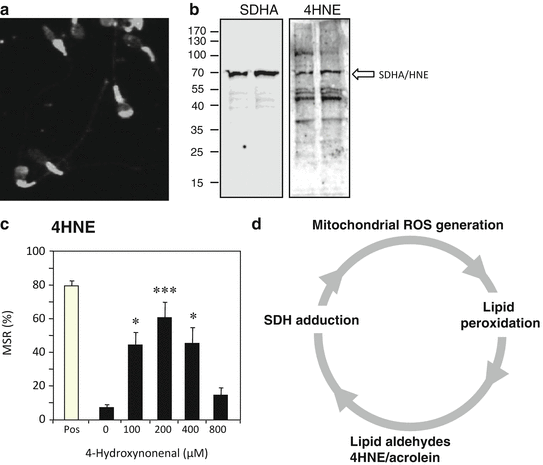
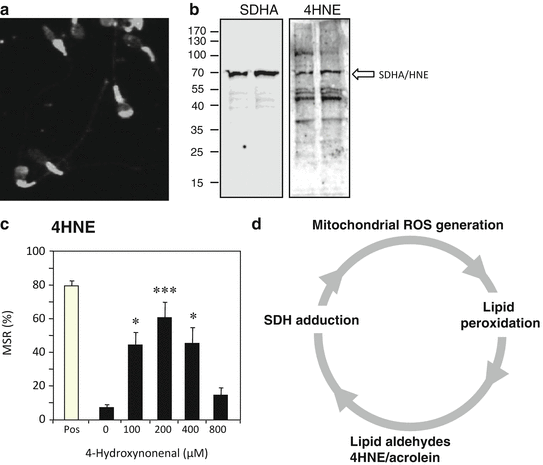
Fig. 2.2
The self-propagating nature of ROS generation by spermatozoa. (a) Immunocytochemical analysis with an anti-4HNE antibody reveals that this electrophilic aldehyde binds to multiple proteins in spermatozoa, particularly in the sperm midpiece where the mitochondria are located. (b) Western blot analysis confirms that multiple proteins are adducted by 4HNE including major proteins from the mitochondrial electron transport chain (ETC) such as succinic acid dehydrogenase (SDHA). (c) The binding of 4HNE to the sperm mitochondria perturbs electron flow through the ETC leading to electron leakage and the generation of ROS; MSR describes the percentage of live cells (defined using SYTOX Green®) that are generating a positive signal with MitoSOX Red ™, a probe for mitochondrial ROS generation, in a flow cytometer. The bell-shaped dose–response curve reflects the ability of 4HNE to compromise cell viability at higher doses (Aitken et al. 2012a, b). (d) As a result of these findings, we propose a self-perpetuating cycle of ROS-induced ROS generation wherein oxidative stress leads to the formation of lipid aldehydes that bind to proteins within the ETC and stimulate yet more ROS generation and lipid peroxidation
2.2.2 Primary Causes of Excess ROS Generation by Sperm Mitochondria
If oxidative stress stimulates a lipid peroxidation process characterized by the generation of aldehydes that perpetuate the generation of ROS from the sperm mitochondria, then the question arises as to what triggers oxidative stress in the first instance. In this context one of the most important activators of ROS generation by sperm mitochondria is the induction of apoptosis in response to senescence or other adverse circumstances.
2.2.2.1 The Intrinsic Apoptotic Cascade in Spermatozoa
One of the most important points to make about apoptosis in spermatozoa is that a regulated, apoptotic cell death is the default position for this cell type and is largely intrinsically induced. To the authors’ knowledge there are no extrinsic factors that will reliably and robustly trigger apoptosis in spermatozoa via a receptor-mediated mechanism. Although powerful bacterially derived factors such as lipopolysaccharide (LPS) have been claimed to trigger apoptosis in human spermatozoa (Hakimi et al. 2006), we have been unable to consistently replicate these findings, despite many attempts. The one condition that will consistently trigger an apoptotic response in these cells is senescence, which is itself a reflection of the oxidative stress created via the formation of lipid aldehydes as a consequence of cell metabolism. If these electrophilic aldehydes are scavenged by powerful nucleophiles such as the thiol, penicillamine (Aitken et al. 2012a, b), then the survival of spermatozoa in vitro is significantly increased. The prolonged life span of mammalian spermatozoa in vivo contrasts dramatically with their limited survival in vitro and suggests that both the male and female reproductive tracts must be capable of controlling the bioavailability of such lipid aldehydes via molecular mechanisms that are still poorly unresolved.
It is the fate of a great majority of spermatozoa to experience a senescence-induced apoptotic death in either the male or female reproductive tract. Whatever the location of this death, its manner is of critical importance. Spermatozoa are terminally differentiated cells that arise long after immunological tolerance has been generated and are potentially immunogenic in both males and females. Thus, when these cells die and are phagocytosed by neutrophils or macrophages, it is important that the phagocytic process responsible for their removal is “silent” and does not trigger a pro-inflammatory, phlogistic response. Such silent phagocytosis occurs commonly in biological systems and is mediated by the phagocyte’s ability to recognize apoptotic markers such as phosphatidylserine and other “eat-me” signals on the exofacial surface of the cell being engulfed (Hochreiter-Hufford and Ravichandran 2013). In order for the massive phagocytic event that follows insemination to be truly silent, it is critical that the spermatozoa undergoing phagocytosis have undergone an apoptotic death. This apoptotic death is generally triggered by the oxidative stress that accompanies the physiological exertion needed to sustain high levels of sperm motility over prolonged periods of time.
It should also be recognized that oxidative stress is an inevitable consequence of the redox mechanisms that are needed to drive the capacitation process forward. “Capacitation” is a general term that covers a range of physiological changes that spermatozoa must undergo if they are to undergo a physiological acrosome reaction in the immediate vicinity of the oocyte. Preparation for acrosomal exocytosis involves a complex array of biochemical changes including cholesterol efflux, a quantum increase in levels of tyrosine phosphorylation, and the expression of hyperactivated movement, all of which are known to be redox regulated (de Lamirande and Gagnon 1993; Aitken et al. 1998; Brouwers et al. 2011). It is theoretically possible for a variety of ROS to be responsible for the induction of these changes associated with capacitation, but the constitutive generation of NO• by spermatozoa suggests that the powerful oxidant, peroxynitrite (ONOO−), is a major product of these cells with a proven capacity to stimulate capacitation (de Lamirande and Lamothe 2009; Rodriguez et al. 2011). If fertilization does not occur, it has been proposed that the continued generation of ONOO− by capacitating spermatozoa leads to a state of “over-capacitation” whereby the ROS generation that drives capacitation eventually overwhelms the limited antioxidant capacity of these cells, leading to a state of oxidative stress (Aitken 2011). The appearance of ONOO−-mediated oxidative stress leads to a loss of sperm function (Uribe et al. 2015) and the generation of lipid aldehydes that via the mechanisms described above, stimulate yet more ROS generation, ultimately precipitating a state of apoptosis characterized by rapid motility loss, mitochondrial ROS generation, caspase activation in the cytosol, annexin V binding to the cell surface, cytoplasmic vacuolization, and oxidative DNA damage (Koppers et al. 2011). During this intrinsic apoptotic cascade, it is only after the spermatozoa have become immobilized, and therefore prone to phagocytosis, that markers of apoptosis such as phosphatidylserine externalization start to appear (Koppers et al. 2011).
Sperm senescence and apoptosis is not normally induced by an external factor, but is an intrinsic process dependent on a fall in phosphoinositide 3-kinase (PI3K) activity. The latter is highly active in mammalian spermatozoa and generates the novel phosphoinositide, PtdIns(3,4,5)P3, which binds to the PH domain of kinases such as AKT1 causing the latter to translocate to the sperm surface where it becomes activated by phosphorylation under the influence of PDK-1. This pathway leads to the promotion of cell survival via a variety of mechanisms, particularly the phosphorylation and inactivation of proapoptotic proteins such as BAD (Koppers et al. 2011). If PI3K activity is suppressed with an inhibitor such as wortmannin, then AKT1 rapidly dephosphoryates, leading, in turn, to the dephosphorylation of BAD and the activation of sperm apoptosis (Koppers et al. 2011). Conversely, any pro-survival factor that stimulates PI3K activity leads to inhibition of apoptosis and prolongs the functional life span of the spermatozoa. There are, in all probability, many such pro-survival factors operating in vivo in order to achieve the prolonged survival of spermatozoa in both the male and female reproductive tracts. One of the first such pro-survival factors to be identified is prolactin (Pujianto et al. 2010). Spermatozoa possess several splice variants of the prolactin receptor, while addition of this hormone to human sperm suspensions has been found to prolong survival in association with an increase in AKT1 phosphorylation (Pujianto et al. 2010).
From the foregoing, it should be clear that oxidative stress is a feature of sperm cell biology responsible for both the induction of sperm capacitation and the eventual demise of these cells as a consequence of a senescence process that is associated with activation of the intrinsic apoptotic cascade. In vitro, sperm senescence and apoptosis are generally promoted by the lack of pro-survival factors in the culture medium. In addition, any factors that promote oxidative stress in spermatozoa have the potential to accelerate the apoptotic process. For example, just exposing spermatozoa to H2O2 will precipitate an apoptotic response (Lozano et al. 2009). Cryostorage of spermatozoa will also generate a high level of apoptosis associated with oxidative stress (Thomson et al. 2009) as will exposure to a number of free radical-generating xenobiotics (Aly 2013) including lifestyle factors such as cigarette smoke and radio-frequency electromagnetic radiation (Fraga et al. 1996; De Iuliis et al. 2009; Liu et al. 2013).
In addition it has been observed that a superabundance of polyunsaturated fatty acids will also stimulate mitochondrial ROS generation and create a state of oxidative stress in human spermatozoa; the greater the level of unsaturation, the greater the stimulatory effect (Koppers et al. 2010). Esterification of the fatty acid counters this prooxidant effect suggesting that it is the amphiphilic properties of these molecules that are central to their ROS-inducing activity, possibly by defining the orientation of the fatty acids in relation to the mitochondrial electron transport chain. In this context, it is significant that defective human spermatozoa generating excessive levels of ROS possess abnormally high cellular contents of free polyunsaturated fatty acids, the levels of which are positively correlated with mitochondrial superoxide generation (Aitken et al. 2006). Why some men should possess spermatozoa generating particularly high levels of ROS in association with high cellular contents of polyunsaturated fatty acids is not currently known; however, such associations may reflect the impact of diet or genetic factors on reproductive function.
2.2.2.2 The Truncated Nature of Apoptosis on Spermatozoa
The reliance of spermatozoa on the intrinsic apoptotic cascade is a distinguishing characteristic of these cells that reflects their status as terminally differentiated, disposable cells. Another unique attribute of apoptosis in spermatozoa is that this process is truncated (Koppers et al. 2011). The reason for such restriction lies in the unique architecture of these cells. Unique among all cell types, spermatozoa are distinguished by the fact that all of the mitochondria and most of the cytoplasm are physically separated from the sperm nucleus (Fig. 2.3). Thus, the conventional apoptosis paradigm, involving nucleases activated in the cytoplasm or released by the mitochondria that move into a centrally located sperm nucleus in order to destroy the DNA and create the cleaved DNA ladders that characterize this process, cannot apply to this cell type. Nucleases generated in the cytoplasm or mitochondria of the sperm midpiece are physically prevented from gaining access to the nuclear DNA located in the sperm head (Koppers et al. 2011). Since chemically, DNA fragmentation can only be induced by free radicals or nucleases, the above rationale explains why most DNA damage in spermatozoa is initially oxidative in nature.
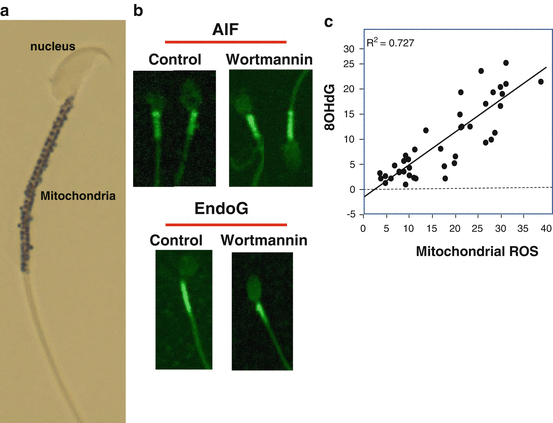
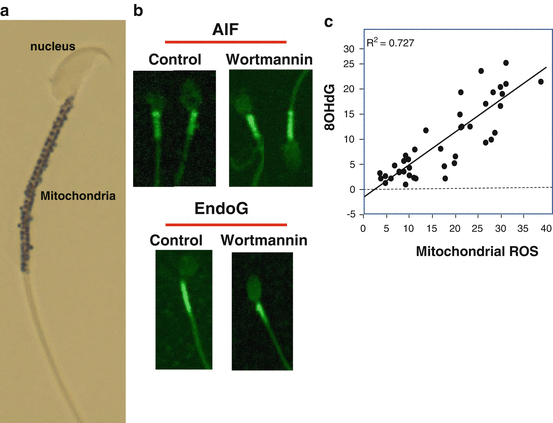
Fig. 2.3
The unique architecture of spermatozoa has an impact on the nature of apoptosis in these cells. (a) Unlike conventional somatic cells spermatozoa are designed in such a way that the sperm nucleus is in a separate physical compartment from all of the mitochondria and most of the cytoplasm. In this image of a mouse spermatozoon, the mitochondrial gyres have been highlighted black using a histochemical stain. (b) As a result of this unusual architecture, apoptosis can be induced with, for example, wortmannin, but nucleases that become activated during this process such as endonuclease G (EndoG) or apoptosis-inducing factor (AIF) remain resolutely locked in the midpiece of the cell and cannot penetrate into the sperm nucleus. This is why most DNA damage in spermatozoa is initiated by oxidative stress rather than intracellular nucleases; the only product of apoptosis that can cross from the midpiece to the sperm head and attack the nuclear DNA is membrane-permeant ROS such as H2O2. (c) As a result of such factors, the release of ROS from the sperm mitochondria is highly correlated with the induction of oxidative DNA damage in these cells
2.2.3 Oxidative Stress in Spermatozoa
High levels of oxidative DNA damage have been repeatedly observed in the spermatozoa of subfertile males (Kodama et al. 1997; Irvine et al. 2000). Oxidative damage occurs primarily at the guanine bases and causes the formation of adducts, the most common of which are 8-hydroxy-2′-deoxyguanosine (8-OHdG) and 8–oxo-7,8-dihydro-2′-deoxyguanosine (8-oxodG), which are commonly used as biomarkers for oxidative stress. Using a flow cytometric assay for 8OHdG, Aitken et al. (2010) demonstrated that the population of males attending an infertility clinic possessed significantly elevated levels of 8OHdG in their spermatozoa compared with an unselected control donor population. The role of ROS in causing such increases in oxidative DNA damage in spermatozoa is suggested by the beneficial impact of antioxidants such as melatonin on the levels of 8OHdG formation observed in human spermatozoa (Bejarano et al. 2014). The source of the ROS responsible for the induction of oxidative DNA damage may be a reflection of three factors: the activation of ROS-generating phagocytic leukocytes, the generation of ROS by the spermatozoa themselves, and a lack of ROS-scavenging enzymes and small molecular mass antioxidants.
2.2.3.1 Leukocytes as a Cause of Oxidative Stress
Infiltrating phagocytic leukocytes are potentially capable of inducing oxidative stress in the male reproductive tract; however, there does not appear to be a strong direct relationship between leukocytospermia and DNA damage in spermatozoa, with data being presented to both support and refute this proposal (Saleh et al. 2002 Moskovtsev et al. 2007). The situation is complex because the degree of oxidative stress experienced by the spermatozoa will depend on the number and type leukocytes present in the ejaculate, when they entered the seminal compartment, whether the leukocytes are activated and, if so, when and how they are activated (Aitken and Baker 1995). Every human ejaculate contains a small number of leukocytes (in the order of 2–5 × 104/ml) that appear to be in a free radical-generating, activated state (Aitken et al. 1995; Aitken and Baker 2013). However, the presence of such leukocytes does not seem to have a powerful effect on sperm function or create particularly high levels of oxidative stress. The reason for this may be that the leukocytes enter the seminal fluid at the level of the secondary sexual glands, particularly when infection is involved. In such instances, the first time the spermatozoa will come into contact with infiltrating leukocytes will be at the moment of ejaculation. At this juncture, the spermatozoa will be protected from extracellular ROS by the powerful antioxidant properties of seminal plasma (Jones et al. 1979; Rhemrev et al. 2000; van Overveld et al. 2000), and the impact of the leukocyte-derived ROS will be minimal. However, quite a different picture emerges when the seminal plasma is removed in the context of preparing spermatozoa for assisted conception therapy. Under these circumstances the protective impact of seminal plasma is lost, and any activated phagocytes that are present in the sperm suspension will have free rein to launch an oxidative attack on the spermatozoa, curtailing the motility of these cells and compromising their DNA integrity. As a consequence of these relationships, negative impacts have been observed between levels of leukocyte contamination in washed sperm preparation and fertilization rates in IVF programs (Krausz et al. 1994).
In order to circumvent such damage occurring during IVF treatment cycles, it is imperative that high-quality spermatozoa are isolated from the ejaculate while these cells are still protected by the antioxidants present in seminal plasma (Aitken and Clarkson 1988). For this reason, procedures such as swim-up from a washed pellet are known to be associated with high levels of oxidative stress and the iatrogenic induction of DNA damage in the spermatozoa (Twigg et al. 1998). One of the best ways to minimize oxidative DNA damage during sperm preparation for IVF is to use a simple, swim-up-from-raw-semen approach (Twigg et al. 1998). Alternative strategies for preparing spermatozoa for assisted conception therapy include the centrifugation of spermatozoa through discontinuous colloidal silicon gradients that isolate the highest quality spermatozoa on the basis of their physical density. Although such techniques are clearly successful in isolating subpopulations of spermatozoa with high levels of motility and good morphology (Aitken and Clarkson 1988), they have been found to actually increase the levels of oxidative DNA damage seen in the spermatozoa (Aitken et al. 2014). The reason for such DNA damage in otherwise high-quality sperm populations has been a mystery until recently, when it was revealed that commercial sperm preparation media are commonly contaminated with metals, including iron and copper, at concentrations that induce high levels of oxidative DNA damage in human spermatozoa (Aitken et al. 2014). Fortunately, the chemical chelation of such metal contaminants is sufficient to eliminate this threat to sperm DNA integrity and render such media suitable for use in an IVF context (Aitken et al. 2014).
2.2.3.2 ROS Generation by Spermatozoa
As indicated above, oxidative stress is commonly caused in spermatozoa by the excess generation of mitochondrial ROS as a consequence of their entry into the intrinsic apoptotic pathway. The induction of mitochondrial ROS generation with, for example, radio-frequency electromagnetic radiation leads to a marked increase in 8OHdG formation followed by DNA fragmentation (De Iuliis et al. 2009). There are many conditions that will trigger the entry of spermatozoa into this pathway including senescence, temperature, exposure to toxic chemicals, and aromatic amino acids (Tosic and Walton 1950; Aitken et al. 2012a, b).
2.2.3.3 Antioxidant Deficiency
Conditions that lead to a loss of antioxidant protection for the spermatozoa such as smoking, poor diet, or prolonged incubation in culture medium lacking antioxidant supplementation can also lead to oxidative stress and oxidative DNA damage in populations of spermatozoa (Fraga et al. 1996; Dalzell et al. 2003; Aitken et al. 2009).
2.2.4 Oxidative DNA Damage in Spermatozoa
In order to explain the etiology of DNA damage in the male germ line, we recently proposed a two-step hypothesis according to which two conditions have to be met in order for such damage to appear: (1) a source of ROS and (2) a state of heightened vulnerability to free radical attack on the part of the spermatozoa. In addition to the presence of oxidizable substrates such as polyunsaturated fatty acids, another key factor defining the vulnerability of spermatozoa to oxidative attack is the status of sperm chromatin. During spermiogenesis there is an extensive remodeling of nuclear chromatin in order to compact the entire haploid genome into the volume of a sperm head. This is achieved by the progressive replacement of nuclear histones with small positively charge proteins known as protamines. In eutherian mammals the proteins have evolved to contain cysteine residues that participate in the creation of intermolecular and intramolecular cross-links during epididymal transit that reinforce the compaction of the chromatin and render the DNA highly resistant to damage. Interestingly, metatherian mammals do not possess cysteine-rich protamines, and as a result their spermatozoa do not undergo this post-testicular phase of nuclear remodeling. As a result, metatherian spermatozoa are much more vulnerable to oxidative DNA damage than their eutherian counterparts (Bennetts and Aitken 2005).
Sperm nuclear DNA is organized into doughnut-shaped toroids linked by nuclease-sensitive interlinker regions, bound to the nuclear matrix, which connect the toroids together (Ward 2010). These interlinker regions are thought to be associated with the retention of histones and to be particularly vulnerable to oxidative attack. Treating sperm with DNase 1 has been found to break apart the toroid linker regions but leaves the DNA within the toroid undamaged. Destruction of the linker regions in this manner has been shown to delay the replication of paternal DNA postfertilization and to impair embryogenesis, suggesting that the DNA contained within these particular regions of sperm chromatin encodes genes that are of critical importance in orchestrating the early stages of embryonic development (Gawecka et al. 2013). Laboratory experiments have demonstrated it is possible to remove protamines and histone-bound nucleosomes by treatment with high salt and reducing agent. This leaves only the sperm nuclear matrix with associated loop domains attached and a resulting nuclear structure that is called a “sperm nuclear halo” (Nadel et al. 1995; Kramer and Krawetz 1996). These halos are useful in giving us an idea as to which part of the chromatin is necessary for the first few cell divisions following fertilization. When sperm halos were injected into oocytes, pronucleus formation was normal and DNA replication was initiated (Shaman et al. 2007). Replication would still occur even if 50 % of the DNA that was not attached to the matrix was removed. As replication will proceed as long as these nuclear matrix-associated regions are present, the interlinker domains must be where DNA replication begins. If this is the case, then oxidative damage to these non-protaminated vulnerable regions of sperm nuclear DNA would be expected to have a major impact on development. In the future, determining which particular genes are housed in these domains would be extremely helpful in understanding how oxidative DNA damage in spermatozoa can influence the developmental potential of the embryo.
Outside of these linker regions, the extent to which sperm DNA becomes protaminated varies between species and between individuals within a species. Thus, in mouse spermatozoa around 95 % of histones are removed and replaced by protamines, whereas in human spermatozoa the equivalent figure is around 15 % (Ward, 2010). Additional vulnerability is created in patient samples because of the inefficient protamination of sperm chromatin. Using the fluorescent probe chromomycin A3 (CMA3) to monitor the degree of sperm chromatin protamination, a very close correlation has been observed between levels of oxidative DNA damage in human spermatozoa and the efficiency of protamine deposition during spermiogenesis; the more poorly protaminated the chromatin, the higher the CMA3 signal and the greater the risk of oxidative DNA damage (De Iuliis et al. 2009).
These observations are important because they add weight to the above mentioned two-step hypothesis of oxidative DNA damage in human spermatozoa. The first step occurs during spermiogenesis and leads to poor protamination of the sperm chromatin, resulting in defective compaction of the DNA and an accompanying increased risk of oxidative attack. The second step then involves the realization of this oxidative attack as a result of the mechanisms described above (Aitken et al. 2009).
Once an oxidative attack on sperm DNA occurs, there are two pathways open to the cell. First, if the oxidative attack is severe, it will initiate the intrinsic apoptotic cascade, culminating in a loss of motility, the appearance of apoptotic markers on the sperm surface, and ultimately cell death. When spermatozoa enter the perimortem and the internal structure of these cells starts to break down, it is possible that sperm nuclear DNA fragmentation becomes accentuated via the activation of intracellular nucleases or the entry of nucleases from the extracellular fluids bathing the spermatozoa in the epididymis or vas deferens (Sotolongo et al. 2005; Boaz et al. 2008; Smith et al. 2013). The purpose of this last-gasp perimortem attack on the sperm nucleus is to facilitate the complete destruction of the sperm genome prior to the phagocytosis of these cells by the immune system. Second, if the spermatozoon is not badly damaged, the oxidative DNA damage may simply be repaired in readiness for fertilization. Counterintuitively it is this process of DNA repair that poses the greatest threat to human health because it is errors in this process that are thought to underpin the connection between oxidative DNA damage in the spermatozoa and the mutational load subsequently carried by children, with important implications for their long-term health trajectory.
2.3 Impacts of Oxidative DNA Damage in Sperm on Human Reproduction and Health
Despite the propensity for human spermatozoa to suffer from oxidative DNA damage, there is relatively little data to confirm that such damage has a major impact on male fertility. The most compelling evidence that this is the case comes from studies in which men exhibiting DNA damage or other evidence of oxidative stress in their spermatozoa have had their sperm DNA integrity or fertility improved by treatment with antioxidants. A study by Suleiman et al. (1996), for example, was distinguished by the careful selection of patients according to evidence of oxidative stress in their spermatozoa, as measured by a lipid peroxidation assay. This trial encouragingly recorded a decrease in lipid peroxidation and a resulting increase in sperm motility and fertility following treatment with vitamin E. Another study selected patients on the basis of DNA damage in their spermatozoa (measured with the TUNEL assay) and recorded a significant improvement in this criterion following treatment with an antioxidant preparation containing vitamin E as well as an accompanying increase in fertility (Greco et al. 2005). These data are certainly promising, but the data sets are too small to draw definitive conclusions about antioxidant therapy and male fertility. There is an urgent need for definitive, randomized, double-blind cross-over trials on this topic to determine the true value of antioxidant therapy in the treatment of males exhibiting high levels of oxidative DNA damage in their spermatozoa (Aitken et al. 2010). The therapeutic efficacy of male antioxidant treatment on the maintenance of subsequent pregnancy has not yet been adequately examined, despite clear evidence linking DNA damage in spermatozoa with an increase in the incidence of miscarriage (Showell et al. 2011; Gharagozloo and Aitken 2011 Robinson et al. 2012).
In order to understand the importance of oxidative DNA damage in spermatozoa on the developmental competence of the embryo and the maintenance of pregnancy, we first need to understand how the 8OHdG lesions formed as a consequence of oxidative stress are repaired in spermatozoa and the zygote.
2.3.1 DNA Repair in Spermatozoa and Early Zygote
Regardless of how well defended the DNA is in sperm chromatin, some form of oxidative damage is inevitable. The base excision repair (BER) pathway plays a vital role in repairing oxidized, alkylated, and deaminated DNA bases and removing small non-helix-distorting base lesions from the genome. In most cells the BER pathway can be broken down into five major steps with each step being performed by a specific enzyme or class of enzymes. The process is highly regulated through individual protein to protein interactions and the formation of repair complexes. Incorporated into the subcellular structure of the sperm nucleus and mitochondria is the first enzyme in the BER pathway, an 8-oxoguanine glycosylase, known as OGG1. When spermatozoa experience an oxidative attack, OGG1 immediately clips the 8OHdG residues out of the DNA generating an abasic site, releasing the oxidized base into the extracellular space (Smith et al. 2013). The next enzyme in the base excision repair pathway, APE1, incises DNA at the phosphate groups, 3′ and 5′ to the baseless site, leaving 3′-OH and 5′-phosphate termini ready for the insertion of a new base. Spermatozoa do not possess this enzyme (Smith et al. 2013). As a result, they carry their abasic sites into the oocyte for continuation of the repair process. Fortunately, the oocyte possesses the remaining elements of the BER pathway including APE1 and XRCC1 (T. Lord and R.J. Aitken, unpublished observations) and is able to carry the repair process through to completion. The repair of oxidative DNA lesions in spermatozoa therefore involves a high level of collision between the male and female germ lines and will be impacted not just by the levels of oxidative stress and 8OHdG formation in the spermatozoa but also the competence of the oocyte to complete the repair process initiated by the spermatozoa. The capacity of the BER pathway in the latter is clearly limited because the spermatozoa of subfertile males are known to carry significantly elevated levels of 8OHdG, which have not been excised by OGG1 (Aitken et al. 2010). It is the responsibility of the oocyte to detect these oxidized base lesions and engage in a round of DNA repair immediately after fertilization and put S-phase on hold until this activity has been completed (Gawecka et al. 2013). Interestingly, although the oocyte possesses an abundance of APE1 and XRCC1, it appears to exhibit a limited supply of OGG1 (T. Lord and R.J. Aitken, unpublished observations). As a result, we can anticipate that 8OHdG adducts will be poorly repaired by the oocyte and may persist into the first cleavage division of the embryo.
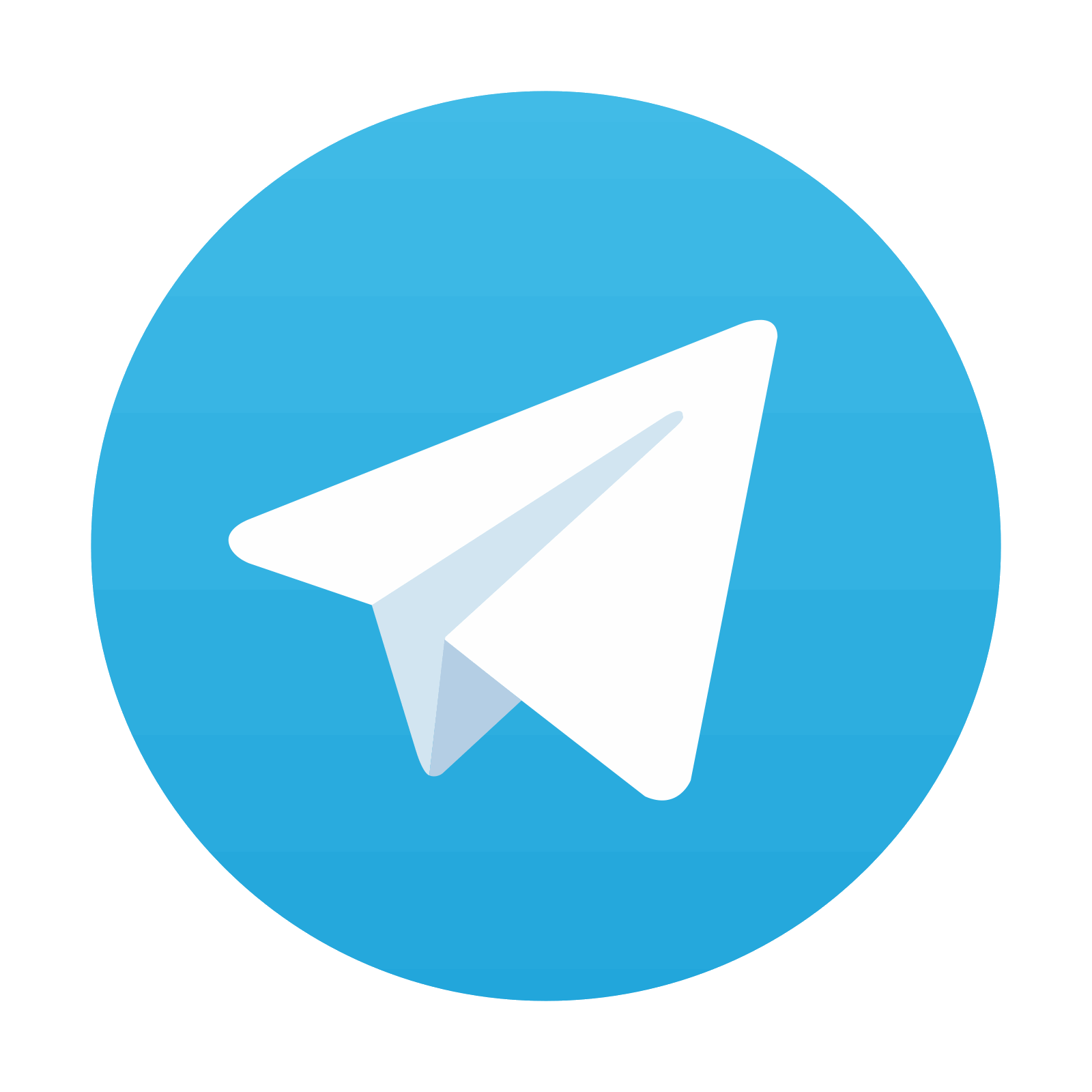
Stay updated, free articles. Join our Telegram channel
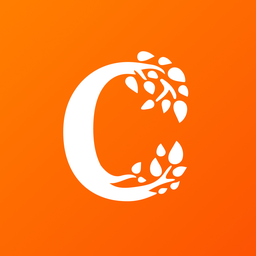
Full access? Get Clinical Tree
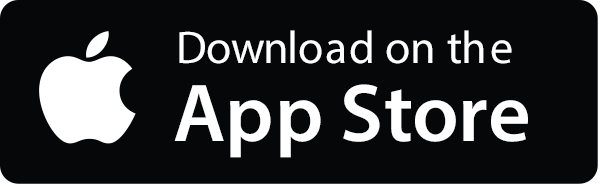
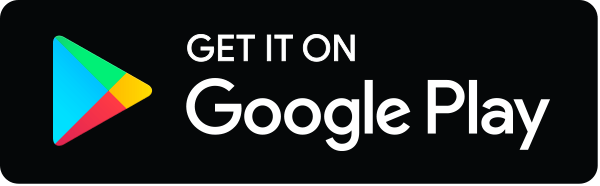