Fig. 12.1
A strategy for constructing engineered tissues (Adapted from Langer and Vacanti [1]). Copyright 1993 AAAS
Various methods have been developed to fabricate cell scaffolds featuring multi-scale structures. To control the multi-scale structures of cell scaffolds, numerous rheological techniques such as the applications of external fields, microfluidic technologies, and self-organizations of biopolymer solutions have been used. In this chapter, several examples of the methods used to control the multi-scale structures of cell scaffolds are described. To design the multi-scale structures of cell scaffolds, understanding how the structure of cell scaffolds affects cellular behaviors is helpful. Therefore, in Sect. 12.2, the effects that microscopic and macroscopic structures of cell scaffolds exert on cellular behaviors are briefly discussed. In Sect. 12.3, we review, also briefly, the materials used to prepare cell scaffolds. In Sects. 12.4 and 12.5, several examples of methods used to control the microscopic and macroscopic structures of cell scaffolds and their applications in the field of tissue engineering are described. In Sect. 12.6, we introduce dialysis-induced anisotropic gelation, which can be used to control both the microscopic and macroscopic structures of cell scaffolds, and then discuss the application of this method in the field of tissue engineering.
12.2 Effects of the Structure and Properties of Cell Scaffolds on Cellular Behaviors
12.2.1 Cell-Extracellular Matrix Interaction
Biological tissues consist of cells and the extracellular matrix (ECM) [2]. The ECM consists of various proteins such as collagen, fibronectin, and laminin, as well as proteoglycans. Therefore, the ECM is regarded as a multicomponent material. Almost all the cells in our body adhere to the ECM, and they do so mainly by means of the interaction between integrin and ECM proteins. Integrin is a transmembrane protein present on the surface of cells that binds to ECM proteins such as collagen, fibronectin, and laminin [3]. The interaction between integrin and ECM proteins translates cues from the ECM into intracellular signal transduction. This adhesion-mediated signal transduction depends on the combinations of integrin and ECM proteins, demonstrating that cells can sense the compositions of the ECM. Therefore, cells behave distinctly depending on the compositions of the ECM. Moreover, cells sense the physical properties of the ECM, such as structural and mechanical properties, through adhesion-mediated signal transduction, and cells respond to these physical properties of the ECM [4–10]. The cell-ECM interaction affects various cellular behaviors such as proliferation, morphology change, adhesion, migration, and differentiation, and thus, the interaction directs the morphogenetic process. Therefore, designing the physical properties of cell scaffolds is crucial for constructing functional engineered tissues.
12.2.2 Effects of the Microscopic Structure of ECM on Cellular Behaviors
Highly ordered structures are often observed in the ECM of connective tissues such as bone, tendon, cornea, and blood vessel. For example, oriented collagen fiber arrays are present in the tendon tissue [11]. The highly ordered structures of the ECM affect not only mechanical properties but also various cellular behaviors in the connective tissues. Pioneering studies on the effects of the ordered structures of ECMs on cell behaviors were conducted by Weiss [4, 12], who showed that cells aligned parallel to the orientation of fibers in blood clots and fish scales (Fig. 12.2). This phenomenon is known as “contact guidance.” Weiss and Taylor also reproduced contact guidance in vitro using glass that had been scored using a MicroLathe [12]: cells elongated along an orientation axis of the microgrooves of the scored glass. Since these seminal studies, the mechanism of contact guidance has been intensively investigated. Although contact guidance has been widely suggested to occur as a result of anisotropic force generation by lamellipodia and filopodia [13, 14], the mechanism of contact guidance remains unresolved. Conversely, contact guidance has been widely reported to induce directional cell migration [15–17]. Directional cell migration is well known to play a key role in tissue morphogenesis [2]. Therefore, the control of contact guidance is crucial when constructing engineered tissues that feature biomimetic hierarchical structures, and hence, methods are required to produce cell scaffolds exhibiting anisotropic structures (anisotropic cell scaffolds) and to control the anisotropic structures of the scaffolds. As introduced in Sect. 12.4, various techniques have been developed to prepare anisotropic cell scaffolds and these techniques have been applied in the field of tissue engineering.


Fig. 12.2
Contact guidance of connective tissue cells cultured on a sheet of parallel collagen fibers obtained from the interior of a fish scale (Adapted from Weiss [12]). Copyright 1959 AIP Publishing LLC
12.2.3 Influence of Macroscopic Structure on the Functions of Tissues
At a macroscopic scale, cell density and morphology and the structure, properties, and compositions of the ECM of native tissues are heterogeneously distributed. The heterogeneities observed in native tissues are spatially regulated and are not completely random. For example, the bone density and the hierarchical structure present at the peripheral parts of long bones are considerably distinct from those at inner parts. Long bones contain cortical bone at the periphery and cancellous bone in the interior. The cortical bone exhibits high bone density and a highly ordered hierarchical structure, whereas the cancellous bone exhibits low bone density and a spongelike structure composed of trabecular bones. The macroscopic inhomogeneity contributes the mechanical properties of long bones [18–20]. By contrast, the confined compression modulus of joint cartilage increases with increasing distance from the articular surface [21]. The compression modulus gradient contributes the functions of cartilage, such as lubrication and shock absorption. Furthermore, the vascular networks present in native tissues consist of vessels of various diameters and lengths and feature self-similar structures [22, 23]. The hierarchical structure of vascular networks results in the efficient transportation of oxygen and nutrients. Thus, mimicking the heterogeneities of tissues is critical for the construction of highly functional engineered tissues.
Tissue morphogenetic processes are controlled through the spatial regulation of cellular functions. Therefore, spatially regulating cellular behavior is crucial for obtaining engineered tissues that mimic the heterogeneities of native tissues. As mentioned in Sect. 12.2.1, cellular behaviors depend on local compositions, structures, and mechanical properties of the ECM. Therefore, controlling the spatial distributions of the compositions, structures, and mechanical properties of cell scaffolds can facilitate the spatial regulation of cellular behavior. For example, culturing cells on a cell scaffold exhibiting an elastic modulus gradient generates a cell density gradient (Fig. 12.3) [24–26]. The formation of a cell density gradient can be attributed to the directional migration of cells induced by the physical cue provided by the elastic modulus gradient of the cell scaffold. This type of directional migration is known as “durotaxis.”


Fig. 12.3
(a) Durotactic behavior of vascular smooth muscle cells cultured on a polyacrylamide hydrogel featuring an elastic modulus gradient. Fluorescent images of F-actin of cells located on the softer region (b), on the intermediate regions (c–d), and on the stiffer region (e) (Adapted from Zaari et al. [26]). Copyright 2004 John Wiley & Sons Inc
In recent years, various methodologies have been developed to control the spatial distribution of compositions, structures, and mechanical properties of cell scaffolds. In Sect. 12.5, several examples of the methods used to regulate the spatial distributions of composition, structure, and mechanical properties of cell scaffolds are presented and the applications of these methods in the field of tissue engineering are described.
12.3 Materials Used for Cell Scaffolds
Cell scaffolds have been produced using various materials such as glass substrates, polymeric solids, and polymer hydrogels. Because using these materials has advantages and disadvantages, we must select the most appropriate materials required to achieve a specified purpose. Here, we briefly review the materials that are commonly used in tissue engineering for the purpose of fabricating cell scaffolds.
12.3.1 Glass Substrates
Glass has been traditionally used as a substrate for culturing cells. Because cells cannot directly adhere to the surface of glass substrates, we must coat the surface of glass substrates with ECM proteins before seeding cells. However, the surface chemical properties of glass substrates can be readily modified using a variety of silane coupling agents. The modified surface of glass substrates can adsorb or bind various biomolecules, allowing the adhesive interactions between cells and the glass substrates to be controlled and the differentiation behaviors of cells to be guided [27].
However, the Young’s modulus of conventional glass substrates is approximately 70 GPa, which is substantially higher than that of bone or teeth, the hardest tissues in our body. Therefore, glass substrates cannot provide biomimetic mechanical microenvironments to cultured cells. Furthermore, glass substrates are not biodegradable and therefore cannot be implanted in the body. Thus, to achieve the goal of tissue engineering, cell scaffolds are required that present highly physiological cultivation conditions and can be implanted in the body.
12.3.2 Polymeric Solids
Polymeric solids have been used most widely as common substrate materials because they can be readily and inexpensively cast into diverse shapes. Surface topographic morphologies of polymeric solids can be modified using various microfabrication techniques such as photolithographic techniques and photoablation [28]. Numerous microfluidic devices have been developed using these microfabrication techniques and used to investigate the microrheological properties of cells and biopolymers [29, 30]. The surface chemical properties of polymeric solids can also be modified using various microfabrication techniques [31, 32]. Recently, several methods to fabricate micro-patterned cell scaffolds have been developed using combinations of modification techniques devised for controlling the surface topographic morphologies and chemical properties of polymeric solids. The topographic effects of cell scaffolds on cellular behaviors such as morphology change and differentiation have been studied by culturing cells on scaffolds that feature micro-patterned surfaces. For example, the mechanism of contact guidance has been intensively investigated using substrates featuring modified surface topographic morphologies and chemistries [7, 13, 14].
Polymeric scaffolds that present macroporous structures, such as sponges and foams, have been prepared using various methods; these scaffolds can be used to culture cells in a 3D environment. Specifically, because macroporous materials are composed of biodegradable polyesters such as polycaprolactone (PCL), polylactic acid (PLA), and poly-3-hydroxybutyrate (P3HB), can be degraded in vivo, and are highly biocompatible, these materials have been used as cell scaffolds to fabricate implantable engineered tissues [33, 34]. The most famous engineered tissue is the earlike engineered tissue that was fabricated by culturing chondrocytes in biodegradable cell scaffolds and then implanting these into the dorsal region of nude mice [35]. Biopolymers such as nucleic acids, proteins, polysaccharides, and proteoglycans can be used as scaffold materials. However, several biopolymers dissolve in water, and biopolymers used as materials for preparing cell scaffolds must be insoluble in water. To make biopolymers insoluble in water, gelation of biopolymer solutions has been often used.
12.3.3 Polymer Hydrogels
A polymer hydrogel consists of a 3D polymer network and water. The polymer hydrogel swells considerably in water and thus exhibits a biomimetic composition of the body. The mechanical properties of polymer hydrogels are similar to those of ECMs in biological tissues, and these properties can be controlled by regulating the cross-linking density of 3D polymer networks. Polymer hydrogels of diverse shapes can be prepared, including spheres, fibers, and disks. As described in this section, various technologies are available for preparing hydrogels featuring anisotropic structures that mimic the ordered structures observed in biological tissues. The characteristics and advantages of polymer hydrogels allow us to investigate various cellular behaviors under biomimetic conditions and to fabricate biomimetic engineered tissues. Numerous polymer hydrogels have been used as cell scaffolds in the fields of cell biology and tissue engineering. Hydrogels composed of biopolymers are especially useful, because these hydrogels are highly biodegradable and biocompatible and they can provide microenvironments to cultured cells that are more physiological than those provided by other types of hydrogels. Furthermore, the toxicities of the degradation products of biopolymer hydrogels, such as oligopeptides and oligosaccharides, are low.
Collagen hydrogels are widely used in the fields of cell biology and tissue engineering because collagen is a major component of the ECM. The collagen molecule can be enzymatically extracted from animal tissues such as bone, tendon, and dermis. The collagen extracted enzymatically is known as atelocollagen, in which the telopeptides at the two terminal regions of native collagen are digested. Because the major antigenic site of collagen is located in the telopeptide, atelocollagen has low antigenicity. Atelocollagen can be dissolved in acidic aqueous solutions such as hydrochloric acid and acetic acid solutions, and atelocollagen hydrogels can be prepared by neutralizing the pH of these solutions. Atelocollagen hydrogels have been traditionally used as a cell scaffold to investigate diverse cellular behaviors. Furthermore, cells can be readily cultured in atelocollagen hydrogels. The behaviors of cells cultured in 3D environments are highly distinct from those of cells cultured in atelocollagen hydrogels [5, 36, 37]. However, atelocollagen hydrogels prepared in accordance with manufactures’ protocols are extremely soft and fragile and exhibit an isotropic structure. Therefore, the handling of atelocollagen hydrogels has been limited, and isotropic atelocollagen hydrogels do not serve as a major template in the construction of engineered tissues featuring biomimetic hierarchical structures.
The composition of Matrigel (or EHS-gel) mimics that of ECMs in native tissues. Matrigel mainly consists of laminin (60 %), Type IV collagen (30 %), and entactin (8 %). The behaviors of cells grown on Matrigel have been determined to be unlike those of cells grown on collagen gels. For example, functions of primary hepatocyte grown on Matrigel, such as albumin secretion, were maintained long term; by comparison, these functions of cells grown on collagen gels declined rapidly [38, 39].
Hydrogels composed of polysaccharides have also been used as cell scaffolds because these are inexpensive to obtain. Alginate is a polysaccharide extracted from brown algae that dissolves readily in water. Alginate hydrogels can be prepared by adding multivalent metal cations such as Ca2+, Mg2+, and Cu2+. Cells cannot directly adhere to the surface of alginate hydrogels. Therefore, biochemical modifications of alginate molecules are required to enable cell-alginate adhesive interactions. Conjugation of the arginine-glycine-aspartic acid (RGD) sequence to alginate molecules is often the biochemical modification performed to mediate cell-alginate adhesive interactions [40–44]. RGD-modified alginate hydrogels have been used to investigate various cellular behaviors in 2D and 3D environments. For example, the adipogenic differentiation of adipose-derived stem cells (ASCs) was enhanced when the cells were cultured in RGD-modified alginate hydrogels [41]. The enhancement of adipogenic differentiation can be attributable to the adhesive interaction between ASCs and the RGD sequence conjugated to the alginate. Moreover, RGD-modified alginate hydrogels can be used as carriers to deliver stem cells [43].
Agarose is a polysaccharide extracted from algae such as Gelidiaceae and Rhodophyta. Agarose dissolves in hot water, and agarose hydrogels are prepared by cooling concentrated agarose solutions. Agarose hydrogels have been frequently used to culture cells in 3D environments. For example, Hanazaki et al. constructed artificial bone tissue by culturing osteoblastic cells in an agarose gel (Fig. 12.4) [44]. Furthermore, agarose hydrogels have been used for investigating the effects of mechanical stimuli on chondrocyte behaviors in 3D environments and for studying the effect of the ECM deposited by chondrocytes on the mechanical properties of agarose hydrogels [45–47].


Fig. 12.4
Engineered bone tissues constructed by culturing MC3T3-E1 cells in agarose hydrogels. The specimens were stained using Alizarin Red S. Arrowheads indicate stained cells in the agarose hydrogel (Adapted from Hanazaki et al. [44])
Biopolymer hydrogels offer several advantages when used for constructing engineered tissues. Specifically, biopolymer hydrogels composed of ECM proteins can mimic cell-ECM interactions in vitro. Therefore, these polymer hydrogels can be regarded as biomimetic cell scaffolds suitable for use in constructing engineered tissues. However, the microscopic structures of biopolymer hydrogels prepared using conventional methods are isotropic, and these hydrogels exhibit homogeneous structures at a macroscopic scale. Therefore, biopolymer hydrogels prepared using conventional methods cannot be used as a template for constructing engineered tissues featuring biomimetic hierarchical structures. Therefore, methodologies that can be used to control the multi-scale structure of biopolymer hydrogel are required for preparing cell scaffolds.
12.4 Control of the Microscopic Structure of Scaffolds and Its Application in Tissue Engineering
As mentioned in Sect. 12.2.2, highly ordered structures are observed in connective tissues, and these structures contribute anisotropic mechanical properties. Therefore, reconstructing the anisotropic structures of native connective tissues is critical for regenerating engineered tissues that possess highly biomimetic structures. Cell scaffolds featuring anisotropic structures (anisotropic cell scaffolds) can be used as a template for constructing engineered tissues that mimic the anisotropic structures of connective tissues. To prepare anisotropic cell scaffolds, controlling the molecular orientation of cell scaffolds is critical. Various techniques have been developed to control the molecular orientation of cell scaffolds. In this section, the methods used to prepare anisotropic cell scaffolds are briefly reviewed.
12.4.1 Isotropic-to-Anisotropic Transitions of Rigid and Semiflexible Biopolymer Solutions
Polymers featuring rigid or semiflexible structures exhibit isotropic-to-anisotropic phase transition. Several biopolymers such as collagen and DNA are regarded as main-chain liquid crystal polymers because these biopolymers possess rigid helical structures. Concentrated solutions of liquid crystal biopolymers exhibit diverse liquid crystalline textures [48–52]. For example, slow evaporation of concentrated DNA solutions generates a liquid crystalline phase that presents a columnar hexagonal structure. These liquid crystalline textures depend on the concentration of DNA molecules and the molecular weight of DNA and on the types of salts added. Similarly, highly concentrated Type I collagen solutions exhibit liquid crystalline phases that mimic the ordered structures observed in the collagen matrix of connective tissues (Fig. 12.5) [51–54]. The morphologies of liquid crystal phases change from a spherulite phase to a dense cholesteric phase when the concentration of collagen is increased [53, 54]. Giraud-Guille and coworkers obtained a dense collagen matrix displaying highly ordered structures by neutralizing the pH of highly concentrated collagen solutions [55] and then used the dense collagen matrix to investigate various properties of fibroblast, such as migration, density, and expression of matrix metalloproteinases, on collagen hydrogels [56]. The fibroblasts seeded on the surface of collagen hydrogels infiltrated the hydrogels to reach deep parts; however, the fibroblasts infiltrated the dense collagen hydrogel considerably more slowly than they infiltrated dilute collagen hydrogels. These results indicate that the dense collagen matrix reduces the infiltration of cells from the surface of the gel to the interior of the gel. By contrast, another group reported that a cholesteric collagen film cast from a dense collagen solution can induce the contact guidance of human fibroblasts [57].


Fig. 12.5
Liquid crystalline phases observed in dense collagen matrices (Adapted from Mosser et al. [53]). Copyright 2006 Elsevier
12.4.2 Flow Field-Induced Orientation of Polymers
External fields such as flow and magnetic fields are tools that can be used to manipulate molecular alignment in scaffolds. When flow fields such as shear flow field and stretched flow field are applied to polymer solutions, polymer chains are deformed and oriented along the direction of the flow field [58–62]. If we apply the flow fields to polymer solutions during the gelation processes, we can obtain hydrogels featuring anisotropic structures. Elsdale and Bard prepared hydrated collagen lattices (HCLs) featuring anisotropic structures by applying a unidirectional drainage flow field to neutralized collagen solutions at semi-dilute concentrations (approximately 0.1 wt%) during the gelation processes [5]. In the anisotropic HCLs, collagen fibers aligned parallel to the drainage direction. Using anisotropic HCLs, the investigators examined the morphology, motility, adhesion, and proliferation of human embryonic lung fibroblasts under 2D and 3D conditions and observed that the fibroblasts aligned parallel to the orientation of the collagen fibers in the HCLs; the orientation of the fibroblasts here could be attributed to contact guidance as described in the preceding paragraph. Dunn and Ebendal prepared anisotropic HCL by using same method and found that chick-heart fibroblasts cultured on wet anisotropic HCL aligned parallel to the orientation of collagen fibers, whereas fibroblasts cultured on air-dried anisotropic HCL did not exhibit contact guidance [6]. These results suggest a key role of 3D culture conditions in the contact guidance of cells. Recently, Lanfer et al. developed a method to finely control the orientation and density of collagen fibrils by combining a microfluidic system and flow field-induced orientation of collagen fibrils [63]; these investigators showed that the degree of collagen fibril orientation and the density of collagen fibrils adsorbed on glass substrates can be controlled by regulating collagen concentration and flow rate and the surface properties of glass substrates, which can be modified by coating with copolymers. Using this anisotropic collagen substrate, Lanfer and coworkers investigated contact guidance and differentiation of human bone marrow-derived mesenchymal stem and progenitor cells (hMSCs) and C2C12 cells (a mouse myoblast cell line) [64] and determined that the myotube assembly of C2C12 cells was regulated by the orientation of collagen fibrils adsorbed on the glass substrate. However, the osteogenic and adipogenic differentiation behaviors of hMSCs cultured on collagen substrates that did or did not feature the anisotropic structure were not markedly different, although contact guidance of the hMSCs was induced on the collagen substrate featuring the anisotropic structure.
12.4.3 Magnetic Field-Induced Orientation of Polymers
Magnetic field can also be used to manipulate the molecular alignment in cell scaffolds. Torbet et al. prepared anisotropic cell scaffolds composed of various fibrous proteins by using a strong magnetic field [65–67]. These investigators showed that the birefringence of the fibrous protein scaffolds, which is related to the degree of fibril orientation, can be controlled by the magnitude of the applied magnetic field. For example, anisotropic collagen hydrogels can be prepared by applying magnetic fields stronger than 1.9 tesla [66]. The contact guidance behaviors of various cells embedded in magnetically aligned collagen scaffolds have been investigated. Guido and Tranquillo described a method to systematically and quantitatively investigate contact guidance behaviors in anisotropic collagen gels prepared by applying magnetic fields [68] and demonstrated that a cell orientation parameter that can be used to quantify cell orientation distribution was linearly proportional to the birefringence intensity of the anisotropic collagen gels. A modified magnetic molecular alignment method was used to prepare an engineered corneal stroma composed of the multilayered collagen gel, in which the orientation of the collagen fibrils changed orthogonally in each layer [69]; keratocytes seeded on the engineered corneal stroma infiltrated the scaffold and the cells aligned parallel to the orientation of the collagen fibrils in each layer.
Anisotropic cell scaffolds prepared using isotropic-to-anisotropic phase transitions exhibit highly ordered microscopic structures that may mimic the microscopic structure of the ECM in connective tissues. Furthermore, because external fields can be applied to finely control molecular alignment in cell scaffolds, the cell scaffolds prepared using flow fields feature well-defined anisotropic structures. These anisotropic cell scaffolds can be used for investigating cellular behaviors under highly physiological conditions and can be used as the template for reconstructing the local anisotropic structures of native tissues. However, because many of the anisotropic cell scaffolds introduced above are homogeneous at a macroscopic scale, they cannot serve as templates that mimic the macroscopic structure of native tissues. Therefore, controlling the macroscopic heterogeneities of cell scaffolds is also crucial.
12.5 Control of the Macroscopic Heterogeneities of Scaffolds and Its Application in Tissue Engineering
As mentioned in the preceding section, at a macroscopic scale, heterogeneous structures are observed in native tissues, and the distribution of these structures is spatially regulated and is not completely random. Such heterogeneous structures could be generated by spatially regulated cellular functions and could contribute the macroscopic functions of native tissues. Therefore, we must regenerate the macroscopic structure of native tissues to obtain highly functional engineered tissues. The spatial distribution of cell density must be controlled and cellular functions must be spatially regulated and, to achieve this, cell scaffolds that feature spatially regulated macroscopic structures are required. To date, numerous methods have been developed to control the macroscopic structures of cell scaffolds, and these methods are briefly reviewed in this section.
12.5.1 Cell Scaffolds Featuring Gradient Properties
As mentioned in Sect. 12.2.2, directional cell migration plays a key role in tissue morphogenesis. The mechanisms that control the direction of cell migration have been intensively investigated in the field of developmental biology. Many of the directional cell migrations observed during development have been widely reported to be regulated by concentration gradients of ligand molecules such as fibroblast growth factors (FGFs), bone morphogenetic proteins, and stromal cell-derived factors. These ligands are known as “chemokines,” and the directional cell migration induced by a concentration gradient of chemokines is called “chemotaxis.” If a concentration gradient of chemokines can be generated in vitro, the morphogenetic processes that occur in vivo could be reproduced. Therefore, controlling chemotaxis in vitro can be considered a critical part of the methodology used to construct engineered tissues featuring biomimetic hierarchical structures. However, because several chemokines are water-soluble biomolecules, maintaining a concentration gradient of chemokines long term is challenging. Hence, techniques are required to immobilize chemokines on cell scaffolds. A polyethylene glycol (PEG) hydrogel featuring a concentration gradient of covalently immobilized FGF was developed using a gradient maker and photopolymerization [70]. On the PEG hydrogel featuring the FGF concentration gradient, vascular smooth muscle cells (VSMCs) aligned parallel to the direction of the concentration gradient and migrated from a region of low FGF concentration to a region of high FGF concentration. Therefore, PEG hydrogels can be used for investigating chemotaxis in vitro, and the technique of immobilizing FGF to generate the concentration gradient can be used to control chemotaxis and cell orientation.
Cells not only sense chemical stimuli by means of specific ligand-receptor interactions but also detect physical stimuli through the adhesive interactions that occur between cells and the ECM. As mentioned in Sect. 12.2.3, cells seeded on cell scaffolds featuring an elastic modulus gradient migrate from soft regions to rigid regions, and this directional cell migration is called “durotaxis.” Therefore, by regulating the spatial distribution of various properties of cell scaffolds, directional cell migration is controlled to construct engineered tissues that exhibit highly biomimetic structures. Numerous cell scaffolds featuring gradients of mechanical properties have been developed to investigate cellular behaviors under physiological conditions in vitro and to test the potential utility of such cell scaffolds in the field of tissue engineering. Microfluidic devices have been frequently used to fabricate cell scaffolds that exhibit gradients of mechanical properties [25, 26].Collagen-coated polyacrylamide hydrogels that exhibit an elastic modulus gradient have been prepared by photopolymerizing precursor solutions under a cross-linker gradient generated using a gradient generator, which was a microfluidic device fabricated using poly(dimethylsiloxane) [71]. VMSCs seeded on this gradient polyacrylamide hydrogel migrated from the soft side to the rigid side. The durotaxis of VMSCs was evaluated using the tactic index developed for a biased persistent random walk, and the durotaxis was shown to increase when the magnitude of the elastic modulus gradient was increased. Moreover, the degree of cell orientation was also reported to increase when the magnitude of the elastic modulus gradient was increased. Thus, cell scaffolds featuring an elastic modulus gradient can control not only the spatial distribution of cells but also cell morphology. Gradient biomaterials composed of ECM proteins are useful for investigating the effects of gradient properties on cellular behaviors under physiological conditions. A 3D collagen gel featuring an elastic modulus gradient was developed using an H-shaped microfluidic device [72] and used to investigate the effect of the elastic modulus gradient on neurite growth; neurite growth was directed and enhanced by the elastic modulus gradient, demonstrating that the 3D collage gel can be used as a substrate to guide the directional regrowth of axons in various nervous systems.
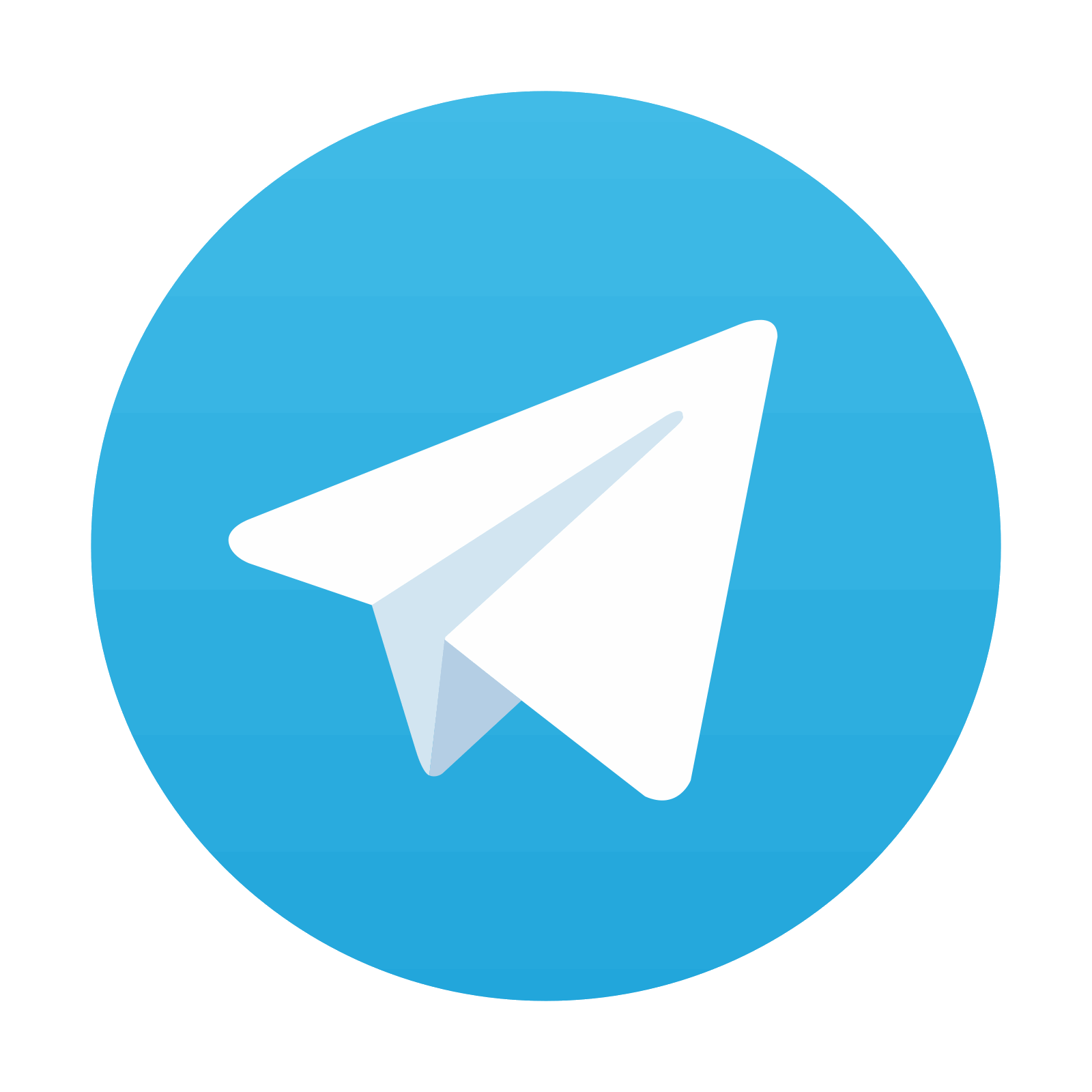
Stay updated, free articles. Join our Telegram channel
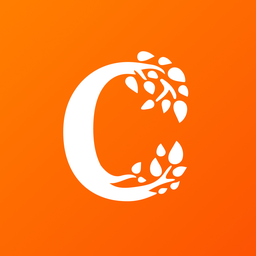
Full access? Get Clinical Tree
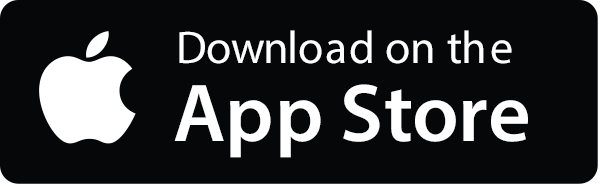
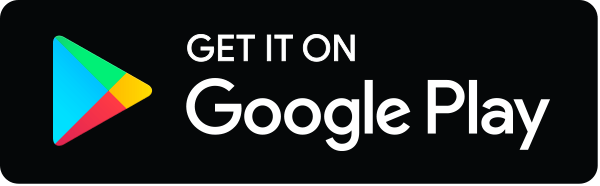