CHAPTER 38 Cellular Motility
Cells move at rates that range over four orders of magnitude (Fig. 38-1 and Table 38-1). At one extreme, ciliates, bacteria, and sperm swim rapidly through water, and giant amoebas crawl rapidly over solid substrates. At the other extreme, fungal, algal, and plant cells with rigid cell walls are immobile. However, even some plant cells move, such as pollen, which extends tubular pseudopods. Most cells, including white blood cells, nerve growth cones, and fibroblasts move at intermediate rates.
Cells produce forces for motility in many different ways, most commonly using the same four mechanisms that produce intracellular movements (see Chapter 37): contraction of actin-myosin networks, movement of motors on microtubules, reversible assembly of actin filaments, or reversible assembly of microtubules. These mechanisms often complement each other, even where movement depends mainly on one system. For example, microtubules contribute to actin-based pseudopod extension by helping to specify the polarity of the cell. The chapter compares these standard mechanisms with a few novel mechanisms: contraction of calcium-sensitive fibers of ciliates, reversible assembly of novel cytoskeletal polymers of nematode sperm, and rotation of bacterial flagellar motors.
Most cells possess the proteins that are required for cellular motility, so the striking variation in their rates of movement arises from differences in the abundance and organization of this machinery. For example, both nonmotile yeasts and contractile muscle cells contain actin, myosin-II, heterodimeric capping protein, α-actinin, and tropomyosin. Yeasts use these proteins for cytokinesis (see Fig. 44-24), while muscle assembles high concentrations of similar proteins into sarcomeres (see Figs. 39-2 and 39-3) for powerful, fast contractions.
Cell Shape Changes Produced by Extension of Surface Processes
Simple alteration of cellular shape can be brought about by assembly of new cytoskeletal polymers or by rearrangement of preexisting assemblies of actin filaments or microtubules. One example that is dependent on assembly of actin filaments is the extension of cell surface projections called filopodia (Fig. 38-2).
Studies of echinoderm sperm revealed that actin polymerization drives the formation of filopodia. Fertilization is accomplished when the sperm extend a long filopodium to penetrate the protective jelly surrounding the egg (Fig. 38-3A). Actin subunits for this acrosomal process are stored with profilin (see Figs. 1-4 and 33-19) in a concentrated packet near the nucleus. Contact with an egg stimulates actin filaments to polymerize, starting from a dense structure near the nucleus. Addition of subunits to the distal (barbed) end of growing filaments drives the elongation of the process and the surrounding membrane at a rate of 5 to 10 μm s−1 (an astounding maximum of 3700 subunits per second). Actin subunits diffuse rapidly enough from their storage site to drive this rapid elongation, which pushes the plasma membrane forward.
Filopodia on macrophages, nerve growth cones (Fig. 38-7), fibroblasts, and epithelial cells grow much more slowly and depend on formins at their tips (Fig. 38-2) to guide barbed-end assembly. Microvilli of the brush border of epithelial cells (see Fig. 33-2) are short, stable filopodia. The bundles of actin filaments supporting microvilli are cross-linked to each other (by fimbrin and villin) and to the plasma membrane by myosin-I. Synthesis of these accessory proteins during embryonic development triggers assembly of microvilli. Similarly, cells with few microvilli can be induced to make more simply by increasing the level of villin.
Sperm of the horseshoe crab, Limulus, use a novel acrosomal process to fertilize an egg (Fig. 38-3B). They preassemble a coiled bundle of actin filaments cross-linked by a protein called scruin. This bundle is a tightly coiled spring. An encounter with an egg stimulates rearrangement of the cross-links, causing the actin bundle to unwind. Uncoiling drives the bundle through a channel in the nucleus followed by extension of a process surrounded by plasma membrane that literally screws its way through the egg jelly to fuse with the egg plasma membrane.
A group of ciliates called heliozoans, named for their similarity to a cartoon of the sun, are unique in using microtubules instead of actin filaments to extend, support, and retract long, thin processes bounded by the plasma membrane (Fig. 38-4). Microtubules in these axopodia are cross-linked into a precise geometrical array that accounts for the rigidity of these long processes. After mechanical stimulation by prey organisms, axopodia collapse in a few seconds, dragging the prey toward the cell body for phagocytosis. The collapse is caused by rapid depolymerization of the microtubules. Ca2+ influx appears to trigger depolymerization of the microtubules, but the details of the mechanism are not known.
Cell Shape Changes Produced by Contraction
Contraction by Actin and Myosin
Cells can change shape by localized or oriented cytoplasmic contractions. Muscle contraction (see Chapter 39) and cytokinesis (see Chapter 44) are the best examples, but contractions remodel many embryonic tissues. Localized contractions at the base or apex of cells in a planar epithelium cause evaginations or invaginations that form the neural tube and glands that bud from the gastrointestinal tract and respiratory tract (Fig. 38-5). Closure of the epidermis over a Drosophila embryo also requires contraction of a circumferential ring of cells. Tension generated by myosin-II and actin filaments deforms each cell and, collectively, the whole epithelium. Similarly, contraction of a ring of actin filaments associated with the zonula adherens of intestinal epithelial cells is one factor regulating the permeability of the tight junctions that seal sheets of epithelial cells (see Fig. 31-2).
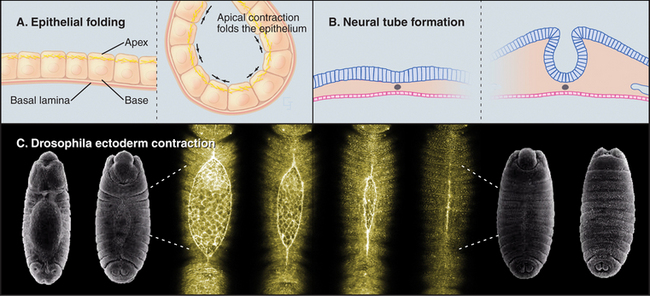
(C, SEMs courtesy of Thom Kaufman, Indiana University, Bloomington [see his movie “Fly Morph-o-genesis” at http://www.sdbonline.org/archive/dbcinema/kaufman/kaufman.html]; light micrographs courtesy of D. Kiehart, Duke University, Durham, North Carolina. Reference: Kiehart DP, Galbraith CG, Edwards KA, et al: Multiple forces contribute to cell sheet morphogenesis for dorsal closure in Drosophila. J Cell Biol 149:471–490, 2000.)
Calcium-Sensitive Contractile Fibers
The ciliate Vorticella avoids predators by contracting a stalk that anchors them to leaves or other supports (Fig. 38-6A). The contractile fibril, called a spasmoneme, contracts faster than any muscle. Ca2+ released from tubular membranes associated with the spasmoneme triggers contractions, when it binds to a calmodulin-like protein, spasmin, that forms 3-nm filaments in the spasmoneme. Ca2+ binding changes the conformation of spasmin and results in rapid shortening, be-cause many spasmin subunits are assembled in series. The spasmoneme relaxes when Ca2+ dissociates. Energy for contraction is supplied indirectly by ATP hydrolysis. ATP-driven pumps create a Ca2+ gradient between the lumen of the membrane system and cytoplasm. Movement of Ca2+ down this gradient drives contraction.
Proteins similar to spasmin are found not only in other ciliates but also in algae, fungi, and animals, where they are called centrin or caltractin. These calmodulin-like proteins form fibrils that anchor centrosomes and the basal bodies of cilia and flagella. Mutations that inactivate caltractin in algae or yeast compromise the duplication and separation of the microtubule organizers (centrosomes or spindle pole bodies; see Figs. 34-16 and 34-19) used for mitosis.
Locomotion by Pseudopod Extension
The ability to crawl over solid substrates or through extracellular matrix is essential for many cells. Perhaps the most spectacular example is the slowly moving growth cone of a nerve axon (Fig. 38-7A). Although moving less than 50 μm s−1, growth cones navigate precisely over distances ranging from micrometers to meters to establish all of the connections in the human nervous system, which consists of billions of neurons and about 1 million miles of cellular processes. Some epithelial cells (Fig. 38-7B) and white blood cells move much faster, about 0.5 μm s−1. These movements enable epithelial cells to cover wounds and allow leukocytes to move from the blood circulation to sites of inflamma-tion (see Fig. 30-13) and to engulf microorganisms by phagocytosis (see Fig. 22-3). During vertebrate embryogenesis, neural crest cells also migrate long distances before differentiating into pigment cells and sympathetic neurons. Fibroblasts lay down collagen fibrils as they move through the extracellular matrix (see Fig. 29-4).
This type of locomotion requires coordination of three different events. The cell must extend its leading edge, adhere to the underlying substrate, and (if the whole cell is to move) retract any attachments of its tail to the substrate. Most cells use assembly of actin filaments to extend pseudopods, but nematode sperm accomplish the same thing with a completely different protein (Fig. 38-11).
Pseudopod Extension
Pseudopods that lead the way in cell migration are filled with a dense, branched network of actin filaments with their fast-growing barbed ends generally facing the plasma membrane (Fig. 38-8). Only the leading lamellum is required for locomotion, since it moves normally after amputation from the rest of the cell (Fig. 38-7B). Generally, the leading lamellum is very flat, on the order of 0.25 μm thick, but some cells extend the lamellum up from the substrate into a wave-like fold of membrane called a ruffle (Fig. 38-2). Microtubules help cells to maintain the polarized shape that is required for persistent directional locomotion, but they are not required for pseudopod extension. A role for actin polymerization in pseudopod extension was originally indicated by the ability of the drug cytochalasin to inhibit the process (see Fig. 33-18).
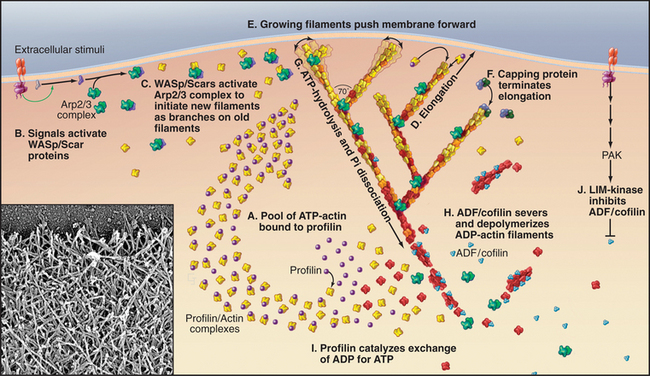
(Redrawn from Pollard TD, Blanchoin L, Mullins RD: Biophysics of actin filament dynamics in nonmuscle cells. Annu Rev Biophys Biomol Struct 29:545–576, 2000, with permission from the Annual Review of Biophysics and Biomolecular Structure, Volume 29, © 2000 by Annual Reviews, www.annualreviews.org. Inset, Courtesy of T. Svitkina and G. Borisy, Northwestern University, Evanston, Illinois.)
Microscopic observations of cells injected with fluorescent actin molecules show that filaments assemble continuously near the leading edge of pseudopods (Fig. 38-9). Purified actin can be labeled with a fluorescent dye and microinjected into live cells, where it incorporates into actin-containing structures, including the cortical network, pseudopods, stress fibers, and surface microspikes. If the dye bound to actin is bleached locally with a strong pulse of light inside a stationary cell (Fig. 38-9A), the bleached spot moves away from the edge of the cell. The spot recovers as bleached actin is replaced with fluorescent actin through a combination of diffusion and active movement of filaments, assembly of new filaments, or subunit flux through filaments. To assess the contribution of each process, a cell can be injected with actin carrying a “caged” dye. The caged dye is not fluorescent until a blocking group is removed locally by photolysis with a pulse of light (Fig. 38-9B). Fluorescent actin monomers diffuse away quickly, so any fluorescent filaments can be observed. In rapidly moving cells, marked filaments remain relatively stationary with respect to the substrate as the front of the cell advances, confirming that new filaments are assembled at the leading edge. The fluorescence of the marked filaments declines over a period of minutes, as fluorescent subunits are released from filaments and diffuse away. In a third approach, a low concentration of fluorescent actin is injected such that it incorporates irregularly into filaments, producing spots of fluorescence that can be tracked over time (see Fig. 33-18). Analysis of these fluorescent speckles confirms that filaments assemble at the leading edge and turn over rapidly deeper in the cytoplasm.
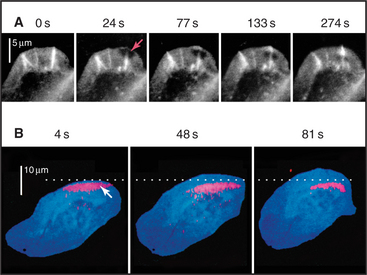
Figure 38-9 documentation of actin filament dynamics at the leading edge with fluorescent actins. A, Fluorescence photobleaching experiment with a stationary cell. Fluorescent actin is injected into a cultured epithelial cell and allowed to incorporate into filaments. A laser pulse bleaches some of the fluorescent actin, leaving a dark spot (arrow) that reveals movement of the filaments toward the cell center. B, Caged fluorescent actin experiment with a motile cell. Fluorescent dye bound to actin is masked with a chemical group preventing fluorescence. After incorporation into actin filaments of a fish keratocyte (see Fig. 33-2E), dyes in one area of the cell are uncaged with a light pulse (arrow), and red fluorescence is followed with time. Fluorescent actin filaments are stationary with respect to the substrate as the cell moves forward (upward). The fluorescent spot of marked filaments fades with time, owing to depolymerization and dispersal of the fluorescent subunits.
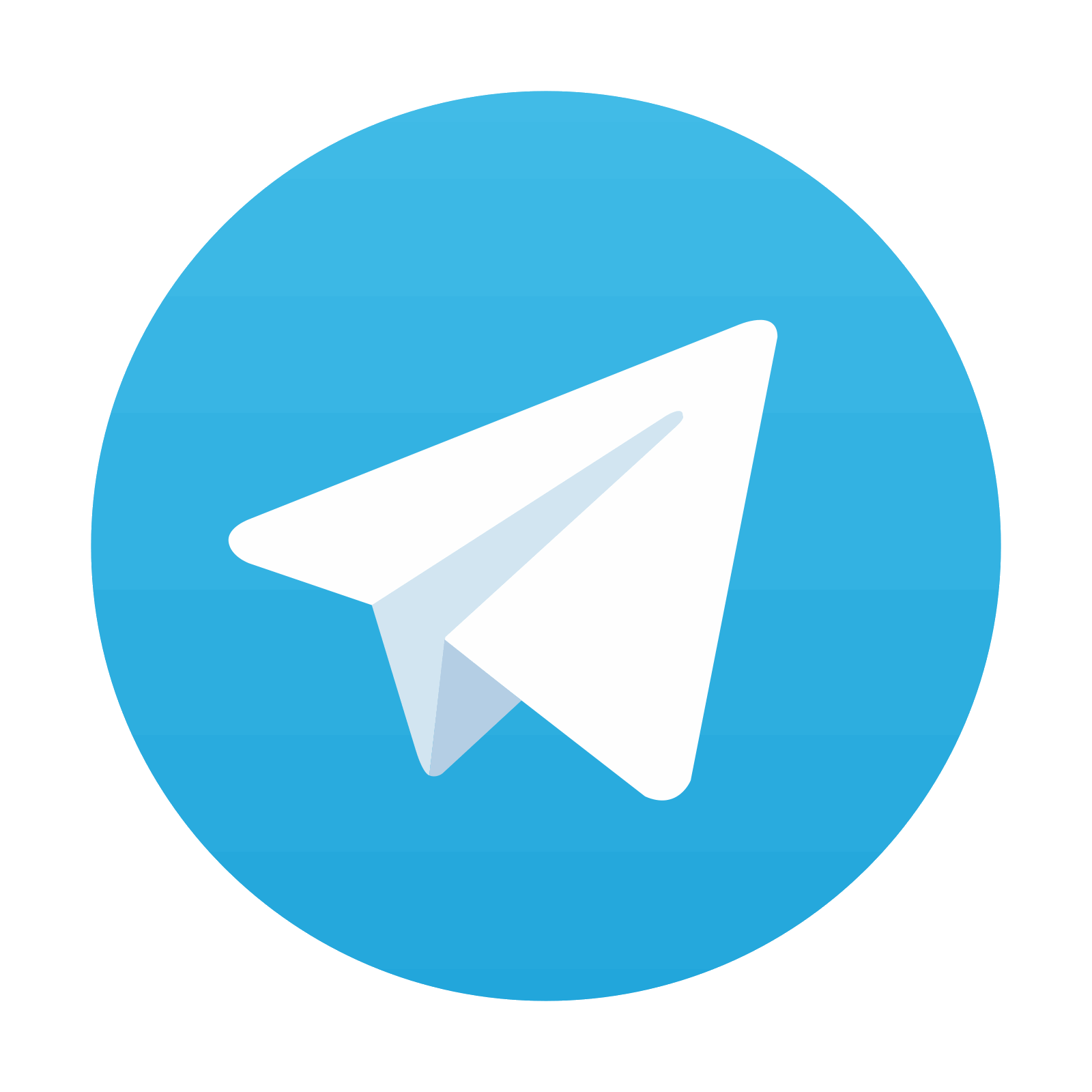
Stay updated, free articles. Join our Telegram channel
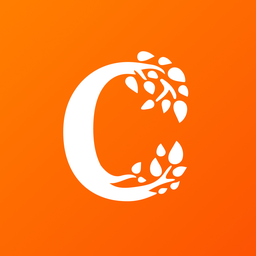
Full access? Get Clinical Tree
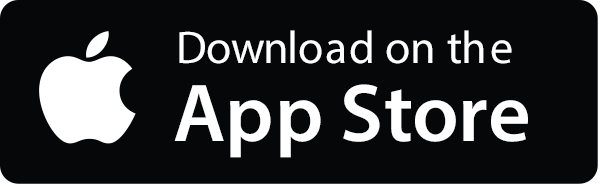
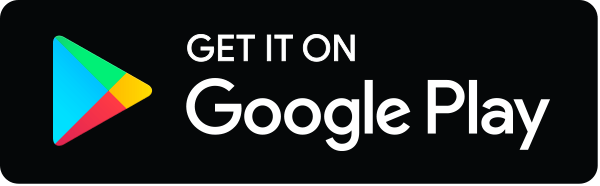