Chapter 1
Cellular Biology
Prokaryotes and Eukaryotes
Besides having structural differences, prokaryotic and eukaryotic cells differ in chemical composition and biochemical activity. The nuclei of prokaryotic cells carry genetic information in a single circular chromosome, and they lack a class of proteins called histones, which in eukaryotic cells bind with deoxyribonucleic acid (DNA) and are involved in the supercoiling of DNA (see Figure 1-2, p. 4). We now understand that the loops and coiling of DNA are important for many diseases (see Chapter 6). Eukaryotic cells have several chromosomes. Protein production, or synthesis, in the two classes of cells also differs because of major structural differences in ribonucleic acid (RNA)–protein complexes. Other distinctions include differences in mechanisms of transport across the outer cellular membrane and differences in enzyme content.
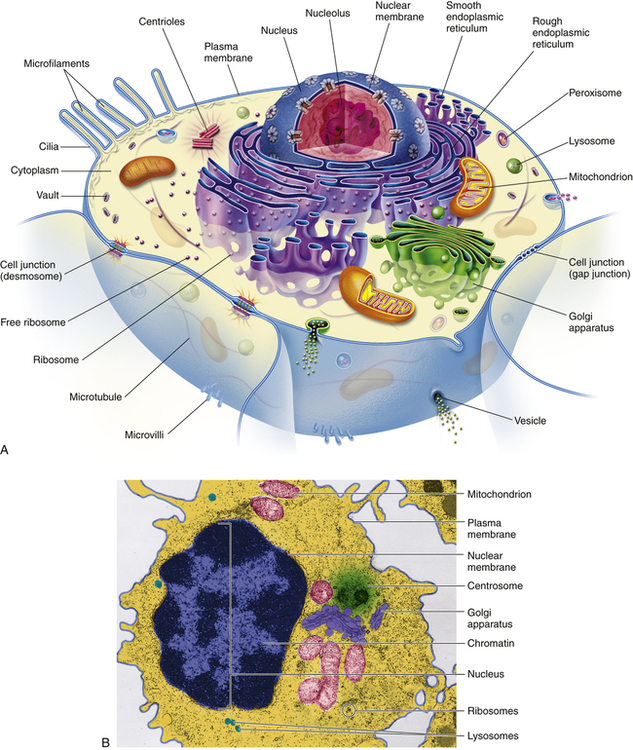
A, Artist’s interpretation of cell structure. B, Color-enhanced electron micrograph of a cell. Both show the many mitochondria known as the “power plants of the cell.” Note, too, the innumerable dots bordering the endoplasmic reticulum. These are ribosomes, the cell’s “protein factories.” (B courtesy of A. Arlan Hinchee. From Patton KT, Thibodeau GA: Anatomy & physiology, ed 8, St Louis, 2013, Mosby.)
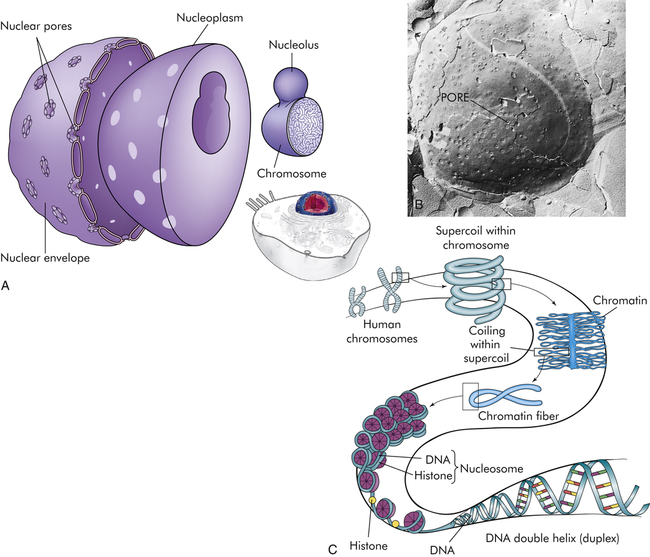
The nucleus is composed of a double membrane, called a nuclear envelope, that encloses the fluid-filled interior, called nucleoplasm. The chromosomes are suspended in the nucleoplasm (illustrated here much larger than actual size to show the tightly packed DNA strands). Swelling at one or more points of the chromosome, shown in A, occurs at a nucleolus where genes are being copied into RNA. The nuclear envelope is studded with pores. B, The pores are visible as dimples in this freeze etch of a nuclear envelope. C, Histone-folding DNA in chromosomes. (B from Raven PH, Johnson GB: Biology, St Louis, 1992, Mosby.)
Cellular Functions
The eight chief cellular functions follow:
1. Movement. Muscle cells can generate forces that produce motion. Muscles that are attached to bones produce limb movements, whereas those that enclose hollow tubes or cavities move or empty contents when they contract. For example, the contraction of smooth muscle cells surrounding blood vessels changes the diameter of the vessels; the contraction of muscles in walls of the urinary bladder expels urine.
2. Conductivity. Conduction as a response to a stimulus is manifested by a wave of excitation, an electrical potential that passes along the surface of the cell to reach its other parts. Conductivity is the chief function of nerve cells.
3. Metabolic absorption. All cells take in and use nutrients and other substances from their surroundings. Cells of the intestine and the kidney are specialized to carry out absorption. Cells of the kidney tubules reabsorb fluids and synthesize proteins. Intestinal epithelial cells reabsorb fluids and synthesize protein enzymes.
4. Secretion. Certain cells, such as mucous gland cells, can synthesize new substances from substances they absorb and then secrete the new substances to serve as needed elsewhere. Cells of the adrenal gland, testis, and ovary can secrete hormonal steroids.
5. Excretion. All cells can rid themselves of waste products resulting from the metabolic breakdown of nutrients. Membrane-bound sacs (lysosomes) within cells contain enzymes that break down, or digest, large molecules, turning them into waste products that are released from the cell.
6. Respiration. Cells absorb oxygen, which is used to transform nutrients into energy in the form of adenosine triphosphate (ATP). Cellular respiration, or oxidation, occurs in organelles called mitochondria.
7. Reproduction. Tissue growth occurs as cells enlarge and reproduce themselves. Even without growth, tissue maintenance requires that new cells be produced to replace cells that are lost normally through cellular death. Not all cells are capable of continuous division (see Chapter 2).
8. Communication. Communication is vital for cells to survive as a society of cells. Pancreatic cells, for instance, secrete and release insulin necessary to signal muscle cells to absorb sugar from the blood for energy. Constant communication allows the maintenance of a dynamic steady state.
Structure and function of cellular components
Figure 1-1 shows a “typical” eukaryotic cell. It consists of three components: an outer membrane called the plasma membrane, or plasmalemma; a fluid filling called cytoplasm; and the intracellular “organs,” or organelles, which are membrane bound and include the nucleus.
Nucleus
The nucleus, which is surrounded by the cytoplasm and generally is located in the center of the cell, is the largest membrane-bound organelle. Two membranes comprise the nuclear envelope (Figure 1-2, A). The outer membrane is continuous with membranes of the endoplasmic reticulum. The inner membrane encloses the neoplasm. The nucleus contains the nucleolus, a small dense structure composed largely of RNA; most of the cellular DNA; and the DNA-binding proteins, the histones, that regulate its activity. The DNA chain in eukaryotic cells is so extensive that the risk of breakage is high. Therefore, the histones that bind to DNA cause DNA to fold into chromosomes (Figure 1-2, C). The wrapping of DNA into tight packages of chromosomes is essential for cell division in eukaryotes.
The primary functions of the nucleus are cell division and control of genetic information. Other functions include the replication and repair of DNA and the transcription of the information stored in DNA. Genetic information is transcribed into RNA, which can be processed into messenger, transport, and ribosomal RNA and introduced into the cytoplasm, where it directs cellular activities. Most of the processing of RNA occurs in the nucleolus. (The role of DNA and RNA in protein synthesis is discussed in Chapter 4.)
Cytoplasmic Organelles
Cytoplasm is an aqueous solution (cytosol) that fills the cytoplasmic matrix—the space between the nuclear envelope and the plasma membrane. The cytosol represents about half the volume of a eukaryotic cell. It contains thousands of enzymes involved in intermediate metabolism and is crowded with ribosomes making proteins. Newly synthesized proteins remain in the cytosol if they lack a signal for transport to a cell organelle.1 The organelles suspended in the cytoplasm are enclosed in biologic membranes, which enables them to simultaneously carry out functions that require different biochemical environments. These functions, many of which are directed by coded messages carried from the nucleus by RNA, include synthesis of proteins and hormones and their transport out of the cell, isolation and elimination of waste products from the cell, metabolic processes, breakdown and disposal of cellular debris and foreign proteins (antigens), and maintenance of cellular structure and motility. Also the cytosol functions as a storage unit for fat, carbohydrate, and secretory vesicles.
Ribosomes
Ribosomes are RNA-protein complexes (nucleoproteins) that are synthesized in the nucleolus and secreted into the cytoplasm through pores in the nuclear envelope called nuclear pore complexes (NPCs).2 These tiny ribosomes may float free in the cytoplasm or attach themselves to the outer membranes of the endoplasmic reticulum (see Figure 1-1, A). Their chief function is to provide sites for cellular protein synthesis. Newly formed ribosomes synthesize a “recognition sequence,” or signal, like an address on a letter. Signal recognition particles (SRPs) in the cytosol bind to the ribosome after recognizing the SRP. Ribophorins, receiver proteins found on the rough sections of the endoplasmic reticulum (ER), act as the “address” site or binding site. The developing protein threads its way through the ER membrane into the lumen. The SRP is removed and the new protein chain is folded into its final conformation.
Endoplasmic Reticulum
The endoplasmic reticulum (ER) (endo = within; plasma = cytoplasm; reticulum = network) is a membrane factory that specializes in the synthesis and transport of the protein and lipid components of most of the cell’s organelles. It consists of a network of tubular or saclike channels (cisternae) that extend throughout the cytoplasm and are continuous with the outer nuclear membrane (Figure 1-3). The folded membranes that form the cisternae of the endoplasmic reticulum may be rough (granular) or smooth (agranular). The rough endoplasmic reticulum (rER) is rough because ribosomes and ribonucleoprotein particles are attached to it (see Figure 1-3). Some of the proteins synthesized by these ribosomes remain in the ER, and others are used to construct membranes of other organelles (the Golgi complex, lysosomes, peroxisomes, and nucleus) and of the cell itself. Importantly, the ER is responsible for much of a cell’s protein synthesis and folding, and a new role is sensing cellular stress (see What’s New? Endoplasmic Reticulum, Protein Folding, and ER Stress). Understanding mechanisms of cellular stress will aid diagnosis and treatment of disease.
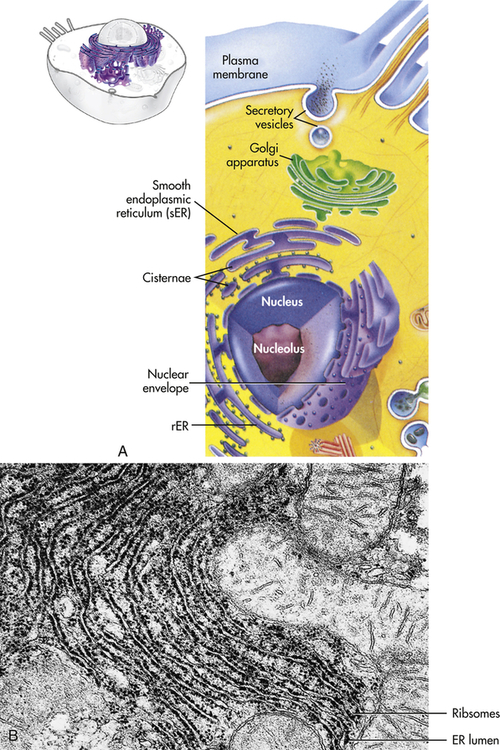
A, The ER consists of rough endoplasmic reticulum (rER) arranged into ribosome-coated cisternae and vesicles of smooth endoplasmic reticulum (sER). B, Electron micrograph of rough and smooth ER. (B courtesy Kelloes C, Farmer M, Center for Advanced Ultrastructural Research, University of Georgia. From Lindsay DT: Functional human anatomy, St Louis, 1996, Mosby.)
Smooth endoplasmic reticulum (sER) does not contain ribosomes or ribonucleoprotein particles (see Figure 1-1). Rather, membranous surfaces of the smooth endoplasmic reticulum contain enzymes involved in the synthesis of steroid hormones and are responsible for a variety of reactions required to remove toxic substances from the cell. The endoplasmic reticulum communicates with the Golgi complex and interacts with other organelles, particularly lysosomes and peroxisomes.
Golgi Complex
The Golgi complex (or Golgi apparatus) is a network of flattened, smooth membranes and vesicles frequently located near the nucleus of the cell (Figure 1-4). Proteins from the endoplasmic reticulum are processed and packaged into small membrane-bound sacs or vesicles called secretory vesicles, which collect at the end of the membranous folds of the Golgi bodies—called cisternae. The secretory vesicles then break off from the Golgi complex and migrate to a variety of intracellular and extracellular destinations, including the plasma membrane. The vesicles fuse with the plasma membrane, and their contents are released from the cell. The best known vesicles are those that have coats made largely of the protein clathrin and are called clathrin-coated vesicles. They bud from the Golgi complex on the outward secretory pathway and from the plasma membrane on the inward endocytotic pathway (see p. 33). Many molecules, including lipids, proteins, glycoproteins, and enzymes of lysosomes, pass through the Golgi complex at some stage in their maturation. The Golgi complex is a refining plant and directs traffic (e.g., protein, polynucleotide, polysaccharide molecules) in the cell1 (Figure 1-5).
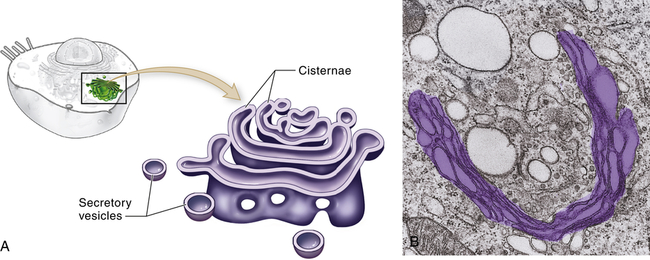
A, Schematic representation of the Golgi complex showing a stack of flattened sacs, or cisternae, and numerous small membranous bubbles, or secretory vesicles. B, Transmission electron micrograph showing the Golgi complex highlighted with color. (From Patton KT, Thibodeau GA: Anatomy & physiology, ed 8, St Louis, 2013, Mosby.)
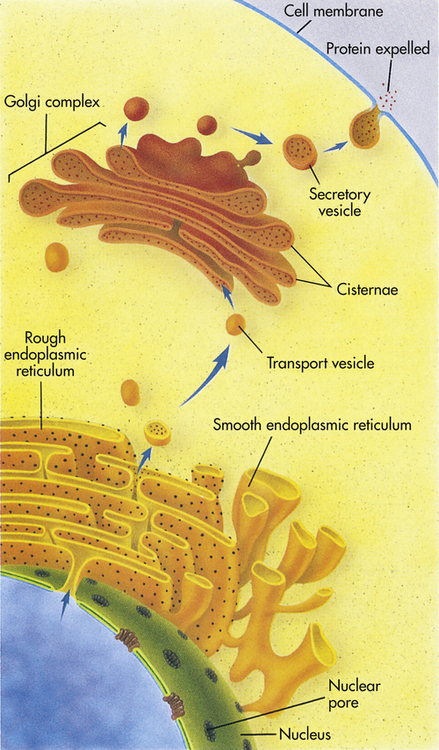
The instructions for making a protein that is destined for export from a cell, such as a digestive enzyme made by a pancreas cell, are first transcribed from DNA by RNA in the nucleus. The RNA then leaves the nucleus through a nuclear pore and proceeds to a ribosome located on the rough endoplasmic reticulum (rER). There it provides instructions for the correct sequence of amino acids for synthesizing that particular digestive enzyme. When enzyme synthesis is complete, the enzyme travels through the ER and is then encapsulated in a transport vesicle. The transport vesicle fuses with a Golgi body, releasing the enzyme. In the Golgi complex the enzyme is further modified and is then shunted to the flattened stacks, or cisternae. There the enzyme waits for a secretory vesicle, which will carry it to the perimeter of the cell, the cell membrane. The secretory vesicle membrane then fuses with the cell membrane, and the enzyme is released outside the cell. (From Raven PH, Johnson GB: Understanding biology, ed 3, Dubuque, IA, 1995, Brown.)
Lysosomes
Lysosomes (lyso = dissolution; soma = body) are saclike structures that originate from the Golgi complex (see Figure 1-1, A). They contain more than 40 digestive enzymes called hydrolases, which catalyze bonds in proteins, lipids, nucleic acids, and carbohydrates. Lysosomes function as the intracellular digestive system (Figure 1-6). Lysosomal enzymes are capable of digesting most cellular constituents completely to their basic components, such as amino acids, fatty acids, and carbohydrates.
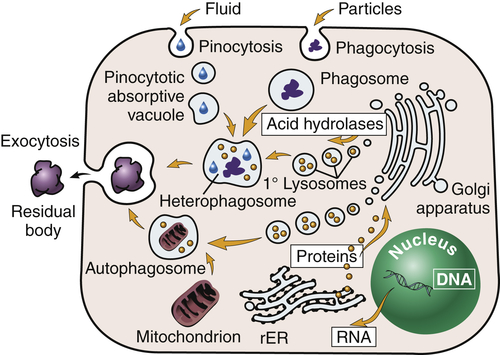
Primary (1°) lysosomes, which originate from the Golgi apparatus, give rise to heterophagosomes and autophagosomes. Undigested material in phagosomes is extruded from the cell or remains in the cytoplasm as lipofuscin-rich residual bodies. rER, Rough endoplasmic reticulum. (From Damjanov I: Pathology for the health professions, ed 4, Philadelphia, 2012, Saunders.)
Lysosomes are necessary for normal digestion of cellular nutrients, intracellular debris, and potentially harmful extracellular substances that must be removed from the body. Extracellular substances are taken into the cell and encapsulated in a membrane-bound vesicle (see discussion on endocytosis, p. 33). Lysosomes merge with the vesicle to form a digestive vacuole. Lysosomes remain fully active by maintaining a low internal pH. They do this by pumping hydrogen ions into their interiors. The hydrolytic enzymes are only maximally active at acid pH values. Lysosomes that are not active do not maintain such an acid internal pH. Lysosomes in this “holding pattern” are called primary lysosomes. When a primary lysosome fuses with a vacuole or other organelle, its pH falls and the hydrolytic enzymes become activated. When it becomes active, it is called a secondary lysosome, or heterophagosome.
As cells complete their life span and die, lysosomes digest the resultant cellular debris. Lysosomes involved in this process, which is called autodigestion, are called autolysosomes, or autophagosomes. In living cells, cellular debris is encapsulated within a vesicle that reacts with a lysosome to complete its degradation. The degradation process, called autophagy, promotes homeostasis because it involves continuous biosynthesis and cell turnover. Recent data indicate that autophagy plays a crucial role in health and disease3,4 (see Chapter 2).
Products of autophagy (and of phagocytosis, the ingestion of harmful foreign substances; see Chapter 7) pass out of the lysosome and are reused by the cell. Indigestible material is stored in vesicles called residual bodies, whose contents are actively expelled from the cell (see Figure 1-6). High concentrations of lipids may accumulate within the residual bodies and remain there for a long time. The lipids are eventually oxidized, and a pigmented substance containing polyunsaturated fatty acids and proteins accumulates in the cell. This pigmented substance, termed lipofuscin, is often called “age pigment” or “age spots,” and is noted in older individuals (see Chapter 2).
Peroxisomes

Thus the breakdown of H2O2 yields H2O and O2 (see discussion of free radicals in Chapter 2). Peroxisomes also have an important role in the synthesis of specialized phospholipids necessary for nerve cell myelination. Such reactions are important in detoxifying various wastes within the cell or foreign components that enter the cell, such as ethanol. Impairment of peroxisomes can lead to disease.
Mitochondria
Mitochondria (mito = thread; chondros = granule), organelles found in large numbers in most cells, are responsible for cellular respiration and energy production (see p. XX). These cytoplasmic organelles appear as spheres, rods, or filamentous bodies that are bound by a double membrane (Figure 1-7). The outer membrane is smooth and surrounds the mitochondrion itself; the inner membrane is convoluted in the mitochondrial matrix to form partitions called cristae. The inner membrane contains the enzymes of the respiratory chain—the name given to the electron-transport chain. These enzymes are essential to the process of oxidative phosphorylation that generates most of the cell’s ATP. Metabolic pathways involved in the metabolism of carbohydrates, lipids, and amino acids and special pathways involving urea and heme synthesis are located in the mitochondrial matrix.
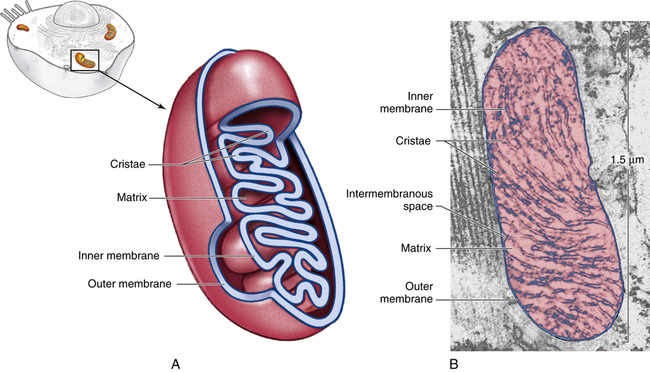
A, Cutaway sketch showing outer and inner membranes. Note the many folds (cristae) of the inner membrane. B, Transmission electron micrograph of a mitochondrion. Although some mitochondria have the capsule shape shown here, many are round or oval. (From Patton KT, Thibodeau GA: Anatomy & physiology, ed 8, St Louis, 2013, Mosby.)
The outer membrane is permeable (passable) to many substances, but the inner membrane is highly selective and contains many transmembranous transport systems. The inner membrane contains a transporter to move electrically charged calcium (calcium ions). (Membrane transport is discussed on p. 25.) Mitochondria contain their own DNA that codes for enzymes needed for oxidative phosphorylation.
Vaults
Vaults are cytoplasmic organelles, also called ribonucleoproteins, that are much larger than ribosomes and shaped like octagonal barrels (Figure 1-8). Their name comes from their multiple arches, which reminded their discoverers of vaulted or cathedral ceilings. A single cell can contain thousands of vaults. The function of vaults is not fully understood and may be related to their octagonal shape. Vaults have a large interior volume and can encapsulate proteins.5 Similarly, the pores in the membrane surrounding the nucleus (see Figure 1-2, B) are also octagonally shaped and the same size as vaults, leading to speculation that vaults may be cellular “trucks.” Further, vaults would dock at nuclear pores, pick up molecules synthesized in the nucleus, and deliver their load elsewhere in the cell. Because at any given time about 5% of the vaults are localized near the nuclear pores, it is thought that vaults may be carrying messenger RNA (mRNA) from the nucleus to the ribosomal sites of protein synthesis within the cytoplasm. Investigators suggest that vaults transport several copies of untranslated RNA and that they are transported along cytoskeletal-based cellular tracks—much like an assembly line.6 Vault complexes are being exploited for the delivery of drugs to the inside of the cell, for example, in kidney diseases.
Cytosol
Excess stored nutrients not immediately used for ATP production are converted in the cytosol into storage forms; for example, excess glucose is stored as glycogen. These temporary masses are known as inclusions (see Chapter 2). Secretory vesicles that have been processed and packaged by the endoplasmic reticulum and Golgi complex also remain in the cytosol. By means of signaling, the vesicles transport and empty their contents outside the cell.
Cytoskeleton
All eukaryotic cells contain elaborate and specialized internal structures in the cytosol that provide the “bones and muscles” of the cell—the cytoskeleton. The cytoskeleton maintains the cell’s shape and internal organization, and it permits movement of substances within the cell and movement of external projections (cilia or microvilli; flagella in sperm) outside the plasma membrane. The cytoskeleton is involved with the extracellular matrix and nuclear interior in force transmission (mechanical forces) called mechanotransduction. Mechanotransduction describes the cellular processes that translate mechanical stimuli into biochemical signals, allowing cells to adapt to their surroundings. Cell stresses, however, that involve alterations of mechanotransduction are associated with several diseases (see Chapter 2).7,8 The internal skeleton is composed of a network of protein filaments; two of the most important are microtubules and actin filaments, or microfilaments.
Microtubules are small, hollow, cylindrical, unbranched tubules made of protein. When found together, microtubules exhibit rigidity, unlike the rest of the cytoplasm. Microtubules thus add strength to the cell’s structure (Figure 1-9, A, B). Within the cell, microtubules support and move organelles from one part of the cytoplasm to another, facilitate transport of impulses along nerve cells, and have roles in the inflammatory and immune responses and hormone secretion (Figure 1-9, C). Microtubules are also involved in the external movement, or motility, of some cells.
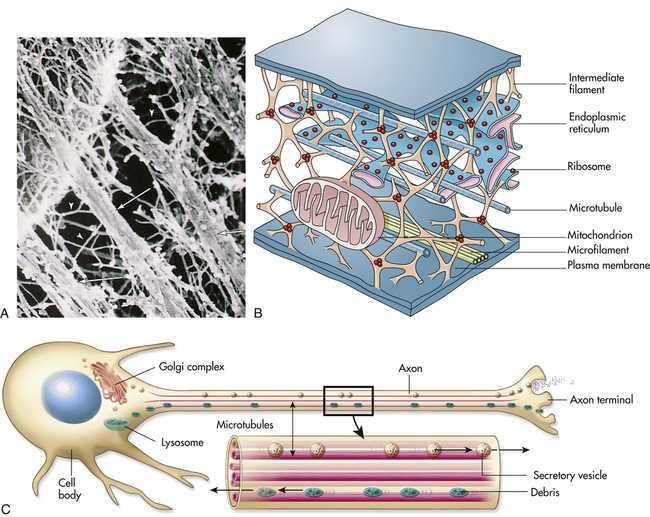
A, Electron micrograph of a portion of the cell’s internal framework. Arrowheads mark the intermediate filaments, and the complete arrows mark the microtubules. B, Artist’s interpretation of the cell’s internal framework. Note that the “free” ribosomes and other organelles are not really free at all. C, Microtubules are necessary for maintaining an asymmetric cell shape, such as that of a nerve cell. In addition, specific chemicals are released from the terminal end of the axon to influence neural transmission. (A and B from Patton KT, Thibodeau GA: Anatomy & physiology, ed 6, St Louis, 2007, Mosby.)
While the cell is not in the process of division, only a few microtubules are assembled; cellular division (mitosis) or defense (phagocytosis) does, however, induce a cycle of rapid assembly and disassembly. Microtubules involved in cellular division are arranged in a centriole. Centrioles always consist of nine bundles containing three microtubules each. During division the pairs of centrioles split and migrate to opposite poles of the cell (see p. 37).
Alterations of microtubular function are implicated in disease processes. For example, alterations in actin microfilaments act as a driving force for cell extension during the dissemination of cancer.9
Actin filaments (microfilaments) are smaller fibrils that generally occur in bundles rather than singly (Figure 1-9, B). Like microtubules, actin filaments are associated with cellular locomotion and maintenance of cell and tissue shape.9 Actin filaments link the interior of the cell to adjacent cells through cell junctions, for example, zonula adherens and zonula occludens (see p. 19).
In addition, microfilaments are necessary for regulating cell growth.10 Cellular locomotion depends on contractile properties that involve both microtubules and actin filaments. The actin cytoskeleton in motile cells has recently been described as a “wave of excitation” that may account for the spontaneous migration of cells.11 Anesthetic drugs can affect both microtubules and actin filaments, disrupting intracellular movement and cellular motility.
Plasma Membranes
Whether they surround the cell or enclose an intracellular organelle, membranes are exceedingly important to normal physiologic function because they control the composition of the space, or compartment, they enclose. Membranes can allow or exclude various molecules, and because of selective transport systems, they can move molecules into or out of the space (Figure 1-10). By controlling the movement of substances from one compartment to another, membranes exert a powerful influence on metabolic pathways. Directional transport is facilitated by polarized domains, distinct apical and basolateral domains. The direction of cells―cell polarity―maintains normal cell and tissue structure for numerous functions, most importantly transport of nutrients in and out of the cell, and becomes altered with diseases, for example, cancer (Figure 1-11). In addition to these functions, the plasma membrane has an important role in cell-to-cell recognition. For example, protein receptors for hormones and for other chemical signals are associated with the membrane and act as markers that identify a cell to its neighbors. Other functions of the plasma membrane include assistance with cellular mobility and maintenance of cellular shape (Table 1-1).
TABLE 1-1
CELLULAR MECHANISM | MEMBRANE FUNCTIONS |
Structure | Usually thicker than the membranes of intracellular organelles Containment of cellular organelles Maintenance of relationship with cytoskeleton, endoplasmic reticulum, and other organelles Outer surfaces in many cells are not smooth but are studded with cilia or even smaller cylindrical projections called microvilli; both are capable of movement; caveolae are also outer indentations Maintenance of fluid and electrolyte balance |
Protection | Barrier to toxic molecules and macromolecules (proteins, nucleic acid, polysaccharides) Barrier to foreign organisms and cells |
Activation of cell | Hormones (regulation of cellular activity) Mitogens (cellular division, see Chapter 4) Antigens (antibody synthesis, see Chapter 8) Growth factors (proliferation and differentiation) |
Transport | Diffusion and exchange diffusion Endocytosis (pinocytosis and phagocytosis); receptor-mediated endocytosis Exocytosis (secretion) Active transport |
Cell-to-cell interaction | Communication and attachment at junctional complexes Symbiotic nutritive relationships Release of enzymes and antibodies to extracellular environment Relationships with extracellular matrix |
Modified from King DW, Fenoglio CM, Lefkowitch JH: General pathology: principles and dynamics, Philadelphia, 1983, Lea & Febiger.
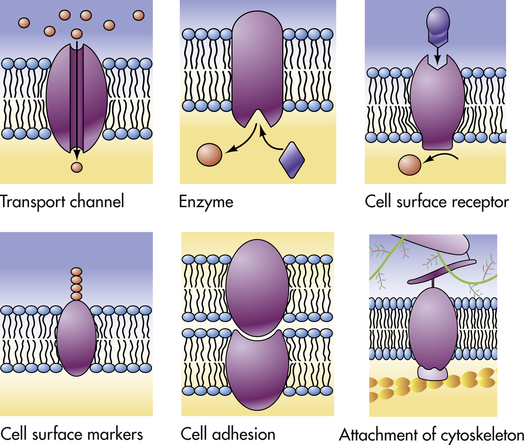
The plasma membrane proteins illustrated here show a variety of functions performed by the different types of plasma membranes. (From Raven PH, Johnson GB: Understanding biology, ed 3, Dubuque, IA, 1995, Brown.)
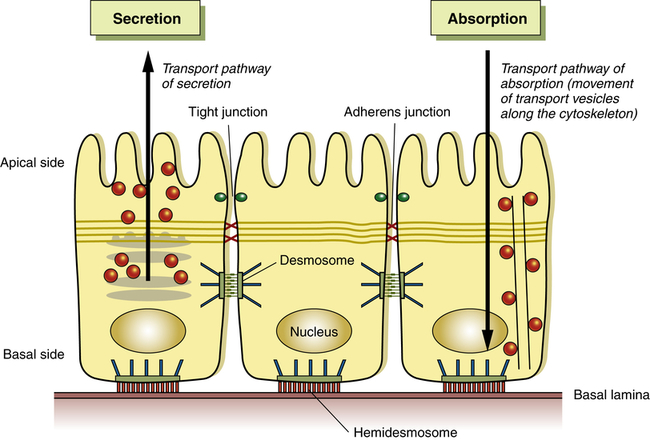
A schematic of cell polarity (cell direction) of epithelial cells. Shown are the direction of the basal side and the apical side. Organelles and cytoskeleton are also arranged directionally to enable, for example, in an intestinal cell secretion and absorption. (Adapted from Life Science web textbook, The University of Tokyo.)
Membrane Composition
Historically, the plasma membrane was described as a fluid lipid bilayer composed of a uniform lipid distribution with inserted moving proteins.12 It now appears that the lipid bilayer is a much more complex structure where lipids and proteins are not uniformly distributed but can separate into discrete units called microdomains, differing in their protein and lipid compositions13 (see What’s New? From the Fluid Mosaic Model to New Understandings about Biologic Membranes). Different membranes have different percentages of lipids and proteins. Intracellular membranes may have a higher percentage of proteins than do plasma membranes, presumably because most enzymatic activity occurs within organelles. The membrane organization is achieved through noncovalent bonds that allow different physical states called phases. The lipid bilayer can be structured into three main phases: a solid gel phase, a fluid liquid-crystalline (or liquid-disordered) phase, and a liquid-ordered phase (see Figure 1-13, p. 14). These phases can change under physiologic factors such as temperature and pressure alterations. The structure of a plasma membrane is shown in Figure 1-12 and Figure 1-13. Intracellular membranes have a higher percentage of proteins than do plasma membranes, presumably because most enzymatic activity occurs within organelles. Carbohydrates are mainly associated with plasma membranes, where they are chemically combined with lipids to form glycolipids and with proteins to form glycoproteins.
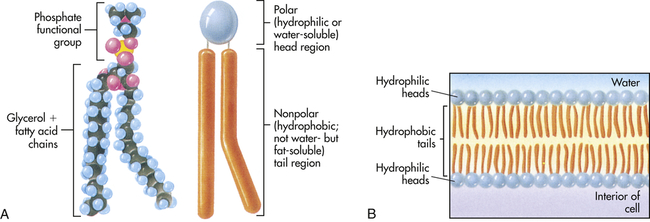
A, Each phospholipid molecule consists of a phosphate functional group and two fatty acid chains attached to a glycerol molecule. B, The fatty acid chains and glycerol form nonpolar, hydrophobic “tails,” and the phosphate functional group forms the polar, hydrophilic “head” of the phospholipid molecule. When placed in water, the hydrophobic tails of the molecule face inward, away from the water, and the hydrophilic head faces outward, toward the water. (From Raven PH, Johnson GB: Understanding biology, ed 3, Dubuque, IA, 1995, Brown.)
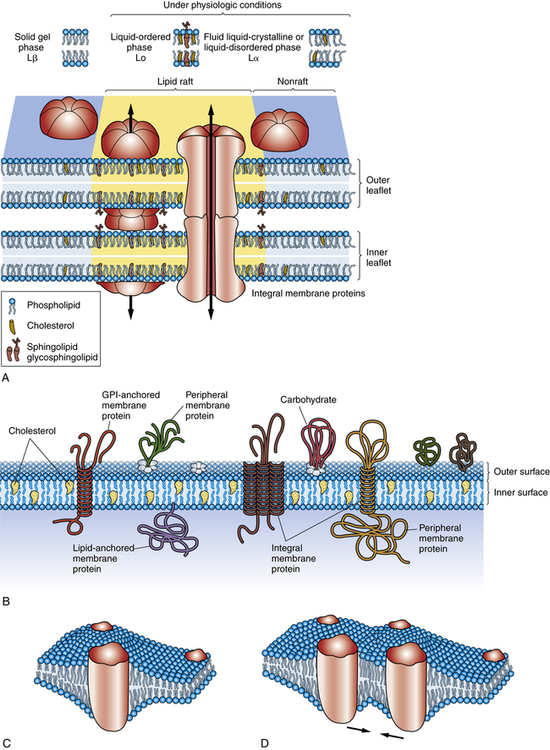
A, Concepts of biologic membranes have markedly changed in the last two decades, from the classic fluid mosaic model to the current model that lipids and proteins are not evenly distributed but can isolate into microdomains, differing in their protein and lipid composition. An example of a microdomain is lipid rafts (colored yellow). Rafts are dynamic domain structures composed of cholesterol, sphinogolipids, and membrane proteins important in different cellular processes. Various models exist to clarify the functions of domains. The three major phases of lipid bilayer organization include a solid gel phase (e.g., with low temperatures), a liquid-ordered phase (high temperatures), and a fluid liquid-crystalline (or liquid-disordered) phase. B, Some membrane-associated proteins are integrated into the lipid bilayer; other proteins are loosely attached to the outer and inner surfaces of the membrane. Transmembrane proteins protrude through the entire outer and inner surfaces of the membrane, and they can be attracted to microdomains through specific interactions with lipids. Interaction of the membrane proteins with distinct lipids depends on the hydrophobic thickness of the membrane, the lateral pressures of the membrane (mechanical force may shift protein channels from an open to closed state), the polarity or electrical charges at the lipid-protein interface, and the presence on the protein side of amino acid side chains. Important for pathophysiology is the proposal that protein-lipid interactions can be critical for correct insertion, folding, and orientation of membrane proteins. For example, diseases related to lipids that interfere with protein folding are becoming more prevalent. C, Investigators are studying the cooperative behavior of lipids, membrane fluctuations, and domains that influence protein organization and consequently protein function. Here a perturbed region develops around an integral protein. D, Two proteins are attracted because of the sharing of a perturbed region of the lipid bilayer. (Adapted from Bagatolli LA et al: Prog Lipid Res 49(4):378–389, 2010; Contreras F-X et al: Cold Spring Harb Perspect Biol 3(6):pii a004705, 2011; Cooper GM: The cell—a molecular approach, ed 2, Washington, DC, 2000; Defamie N, Mesnil M: Biochim Biophys Acta, 2011 Oct 2. [Epub ahead of print.]. )
The outer surface of the plasma membrane in many types of cells, especially endothelial and adipocytes, is not smooth but dimpled with cavelike indentations known as caveolae (“tiny caves”). Caveolae and caveolae-like domains are structurally heterogeneous.13 Caveolae were considered to be functionally insignificant until the mid-1990s, when evidence suggested that they (1) serve as a repository for some receptors, (2) provide a new route for transport into the cell, and (3) act as the initiator for relaying signals from several extracellular chemical messengers into the cell’s interior14 (see p. 20).
Lipids
A major component of the plasma membrane is a bilayer of lipid molecules. It is now known that membranes include up to a thousand lipids that vary in structure.15 The wide range of lipids is thought to be the solvent for membrane proteins.13 Lipids along with protein assemblies act as “molecular glue” for the structural integrity of the membrane. Each lipid molecule is said to be polar, or amphipathic. An amphipathic molecule is one in which one part is hydrophobic (uncharged, or “water hating”) and another part is hydrophilic (charged, or “water loving”) (see Figure 1-12). The membrane spontaneously organizes itself into a bilayer because of these two incompatible solubilities. The hydrophobic region (hydrophobic tail) of each lipid molecule is protected from water, whereas the hydrophilic region (hydrophilic head) is immersed in it. The bilayer’s structure accounts for one of the essential functions of the plasma membrane: it is impermeable to most water-soluble molecules (molecules that dissolve in water) because they are insoluble in the oily core region. The bilayer serves as a barrier to the diffusion of water and hydrophilic substances while allowing lipid-soluble molecules, such as oxygen (O2) and carbon dioxide (CO2), to diffuse through it readily. Because the bilayer is fluid at temperatures above freezing, components of the cellular environment move slowly and selectively across the membrane all the time. (Components of the cellular environment are also discussed in Chapter 3.)
Some membranes contain certain lipids that organize into lipid rafts. Lipid rafts consist of cholesterol and sphingolipid-dependent microdomains that form a network of lipid-lipid, protein-protein, and protein-lipid interactions (Figure 1-13). Lipid rafts may have several functions including the segregation of signaling mechanisms and molecules, the control of cytoskeletal remodeling events, and the targeted movement of associated proteins.16
Proteins
A protein is made from a chain of amino acids, known as polypeptides. There are 20 types of amino acids in proteins and each type of protein has a unique sequence of amino acids. Proteins are the major workhorses of the cell. After translation of a protein, posttranslational modifications (PTMs) are the methods used to diversify the limited numbers of proteins generated. These modifications alter the activities and functions of proteins and have become very important in understanding diseases. Researchers have known for decades that pathogens interfere with the host’s PTMs.17 New approaches are being used to understand changes in proteins—a field called proteomics is the study of the proteome or entire set of proteins expressed by a genome from synthesis, translocation, and modification (e.g., folding) and their role in a staggering number of diseases (also see What’s New? Endoplasmic Reticulum, Protein Folding, and ER Stress, p. 6).
Although the classification of membrane proteins as peripheral or integral is commonly used, it does not describe how proteins are associated with the bilayer. Another mode of classification does so by taking into account the membrane-spanning, or transmembranous, nature of membrane proteins1 (see Figure 1-13, A). According to this classification, proteins are associated with the lipid bilayer in four ways:
1. Some proteins, called transmembrane proteins, extend across the bilayer and are exposed to an aqueous environment on both sides.
2. Some intracellular proteins extend their polypeptide chain partially through the bilayer by means of a fatty acid chain.
3. Some cell surface proteins are attached to the bilayer by a covalent linkage (i.e., a specific oligosaccharide).
4. Some proteins do not extend even partially through the bilayer but are bound to the membrane by noncovalent linkages with other membrane proteins.
Proteins exist in densely folded molecular configurations rather than straight chains; so an excess of hydrophilic units is at the surface of the molecule and an excess of hydrophobic units is inside. Membrane proteins like other proteins are synthesized by the ribosome and then travel, called trafficking, to different membrane locations of a cell.18 Trafficking places unique demands on membrane proteins for folding, translocation, and stability.18 Thus, much research is now being done to understand misfolded proteins, for example, as a cause of disease (see What’s New? Endoplasmic Reticulum, Protein Folding, and ER Stress, p. 6).
Proteins facilitate transport across membranes by serving as receptors, enzymes, or transporters. Proteins act as: (1) recognition and binding units (receptors) for substances moving in and out of the cell; (2) pores or transport channels for various electrically charged particles called ions or electrolytes and specific carriers for amino acids and monosaccharides; (3) specific enzymes that drive active pumps that promote concentration of certain ions, particularly potassium (K+), within the cell while keeping concentrations of other ions, for example, sodium (Na+), below concentrations found in the extracellular environment; (4) cell surface markers, such as glycoproteins (proteins attached to carbohydrates) that identify a cell to its neighbor; (5) cell adhesion molecules (CAMs), or proteins that allow cells to hook together and form attachments to the cytoskeleton for maintaining cellular shape; and (6) catalysts of chemical reactions, for example, conversion of lactose to glucose. (Membrane transport is discussed on p. 28.) Membrane proteins are key components of energy transduction, converting chemical energy into electrical energy, or electrical energy into either mechanical energy or synthesis of ATP.18
Proteolytic Cascades
Proteases are enzymes that cause the breakdown of proteins. Certain proteases can be tethered to cell membranes. Proteases are involved in the physiologic regulation of essential processes by participating in a tightly orchestrated sequence of events termed a proteolytic cascade. Four major proteolytic cascades with disease relevance are candidates for treatment modalities, including (1) cell death or caspase-mediated apoptosis, (2) blood coagulation cascade, (3) degrading membrane enzymes or matrix metalloproteinase cascade, and (4) the complement cascade. Some proteases within a proteolytic cascade act as initiators; others are involved in amplification and propagation and execution (Figure 1-14). Understanding the various steps involved is crucial for designing drug interventions. Dysregulation of proteases features prominently in many human diseases, including cancer, autoimmunity, and neurodegenerative disorders.19–21
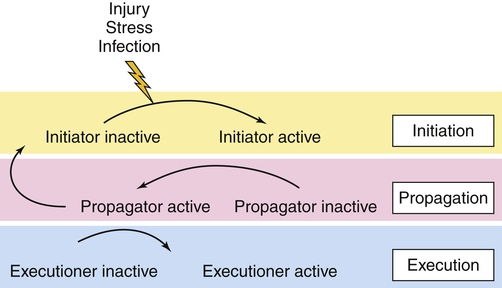
In the initiation phase, the cascade is triggered by an external stimulus, such as injury, stress, or infection. During the propagation phase, the initiator converts a downstream propagator into its active form by proteolysis. In the execution phase, the propagator will activate an executor. The process of coagulation is the best known proteolytic cascade. (Redrawn with permission from Amour A et al: Biochem Soc Trans 32:15–16, 2004. © The Biochemical Society.)
Cellular Receptors
Cellular receptors are protein molecules (proteins are discussed on p. 13) on the plasma membrane, in the cytoplasm, or in the nucleus that are capable of recognizing and binding with specific smaller molecules called ligands (Figure 1-15). Hormones, for example, are ligands. Recognition and binding depend on the chemical configuration of the receptor and its smaller ligand, which must fit together somewhat like pieces of a jigsaw puzzle (see Chapter 21). Activation of a receptor also may depend on differences in movement and binding of the extracellular face of the receptor.22
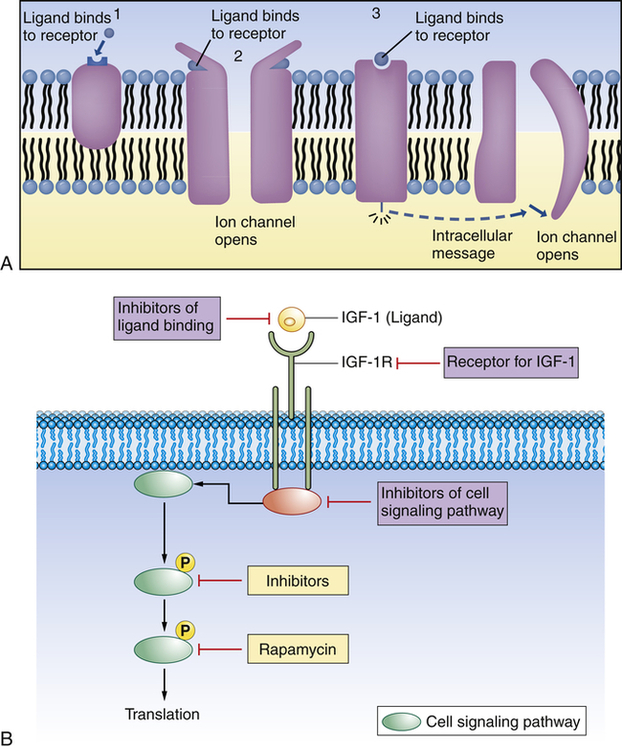
(A) 1, Plasma membrane receptor for a ligand (here, a hormone molecule) on the surface of an integral protein. A neurotransmitter can exert its effect on a postsynaptic cell by means of two fundamentally different types of receptor proteins: 2, channel-linked receptors, and 3, non–channel-linked receptors. Channel-linked receptors are also known as ligand-gated channels. (B) Example of ligand-receptor interaction. Insulin-like growth factor 1 (IGF-1) is a ligand and binds to the insulin-like growth factor 1 receptor (IGF-1R). With binding at the cell membrane the intracellular signaling pathway is activated, causing translation of new proteins to act as intracellular communicators. This pathway is important for cancer growth. Researchers are developing pharmacologic strategies to reduce signaling at and downstream of the IGF-1R, hoping this will lead to compounds useful in cancer treatment.
Plasma membrane receptors are particularly important for cellular uptake of ligands (see Figure 1-15, B, and Table 1-2). They protrude from or are exposed at the external surface of the membrane and often are attached to integral proteins. Some of these recognition units have all the mobile properties related to membrane fluidity. The ligands that bind with membrane receptors include hormones, neurotransmitters, antigens, complement components, lipoproteins, infectious agents, drugs, and metabolites. The past several years have brought many new discoveries concerning the specific interactions of cellular receptors with their respective ligands. In many instances this information has provided a basis for understanding disease.
TABLE 1-2
CLASSES OF PLASMA MEMBRANE RECEPTORS
TYPE OF RECEPTOR | DESCRIPTION |
Channel linked | Also called ligand-gated channels; involve rapid synaptic signaling between electrically excitable cells. Channels open and close briefly in response to neurotransmitters, changing ion permeability of plasma membrane of postsynaptic cell. |
Catalytic | Once activated by ligands, function directly as enzymes. Composed of transmembrane proteins that function intracellularly as tyrosine-specific protein kinases. |
G-protein linked | Indirectly activate or inactivate plasma membrane enzyme or ion channel; interaction mediated by guanosine triphosphate (GTP)–binding regulatory protein (G protein). When activated, a chain of reactions occurs that alters concentration of intracellular messengers, such as cyclic adenosine monophosphate (cAMP) and calcium, or signaling molecules. Behaviors of other target proteins are also altered. May also interact with inositol phospholipids, which are significant in cell signaling, and molecules involved in the inositol-phospholipid transduction pathway. A G-protein–linked receptor activates the enzyme phosphoinositide-specific phospholipase, which in turn generates two intracellular messengers: (1) inositol triphosphate (IP3) releases Ca++, and (2) diacylglycerol remains in the plasma membrane and activates protein kinase C. Protein kinase C further activates various cell proteins. Several different plasma membrane receptors are known to use the inositol-phospholipid transduction pathway. |
Data from Alberts B et al: Molecular biology of the cell, ed 5, New York, 2008, Garland.
Although the chemical nature of both ligands and the receptors to which they bind differs, receptors are classified on the basis of their location and function (see Cellular Communication and Signal Transduction, p. 20). Cellular type determines overall cellular function, but plasma membrane receptors determine which ligands a cell will bind with and how the cell will respond to binding with each. For example, the ability of a hormone or a neurotransmitter to stimulate a cell is regulated by the specificity and number of receptors present on the plasma membrane. Specific processes also control intracellular mechanisms. Hormone binding, for example, depends on special messenger molecules that regulate protein synthesis within the cell (see Chapter 21). Neurotransmitters (discussed in Chapter 15) also operate by causing special messengers to react with specific receptors.
Receptors for different drugs are found on the plasma membrane, in the cytoplasm, and in the nucleus. Membrane receptors have been found for certain anesthetics, opiates, endorphins, enkephalins, antibiotics, cancer chemotherapeutic agents, digitalis, and other drugs. Membrane receptors for endorphins, which are opiate-like peptides isolated from the pituitary gland, are found in large quantities in pain pathways of the nervous system (see Chapters 15 and 16). With binding, the endorphins (or drugs like morphine) change the cell’s permeability to ions, increase the concentration of molecules that regulate intracellular protein synthesis, and initiate molecular events that modulate pain perception.
Receptors for infectious microorganisms, or antigen receptors, bind bacteria, viruses, and parasites. Antigen receptors on white blood cells (lymphocytes, monocytes, macrophages, granulocytes) recognize and bind with antigenic microorganisms and activate the immune and inflammatory responses (see Chapters 7 and 8).
Cell-to-Cell Adhesions
Extracellular Matrix
Cells can be bound together by attachment to one another or via the extracellular matrix (also including the basement membrane), which the cells secrete around themselves. The extracellular matrix is an intricate meshwork of fibrous proteins embedded in a watery, gel-like substance composed of complex carbohydrates (Figure 1-16). The matrix is like glue; however, it does provide a pathway for diffusion of nutrients, wastes, and other water-soluble traffic between the blood and tissue cells. Interwoven within the matrix are three groups of macromolecules: (1) fibrous structural proteins, including collagen and elastin; (2) a diverse group of adhesive glycoproteins, such as fibronectin; and (3) proteoglycans and hyaluronic acid. The basement membrane is a thin layer of connective tissue underlying the epithelium of many organs and is also called the basal lamina (see Figure 1-16).
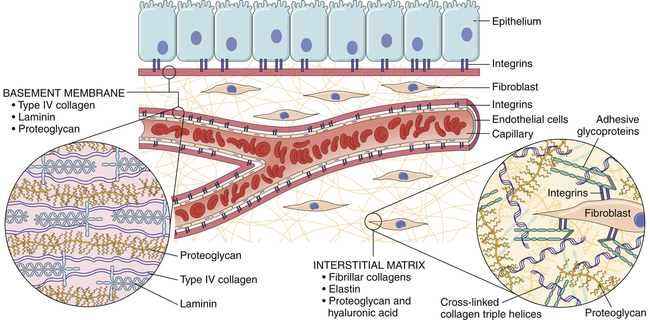
Tissues are not just cells but also extracellular space. The extracellular space is an intricate network of macromolecules called the extracellular matrix (ECM). The macromolecules that constitute the ECM are secreted locally (by mostly fibroblasts) and assembled into a meshwork in close association with the surface of the cell that produced them. Two main classes of macromolecules include proteoglycans, which are bound to polysaccharide chains called glycosaminoglycans; and fibrous proteins (e.g., collagen, elastin, fibronectin, and laminin), which have structural and adhesive properties. Together the proteoglycan molecules form a gel-like ground substance in which the fibrous proteins are embedded. The gel permits rapid diffusion of nutrients, metabolites, and hormones between the blood and the tissue cells. Matrix proteins modulate cell-matrix interactions including normal tissue remodeling (which can become abnormal, for example, with chronic inflammation), embryogenesis, wound healing, and angiogenesis. Disruption of this balance results in serious diseases such as arthritis, tumor growth, and others. (From Kumar V et al: Robbins & Cotran pathologic basis of disease, ed 8, Philadelphia, 2010, Saunders.)
All of these macromolecules occur in intercellular junctions and cell surfaces and may assemble into two different components: interstitial matrix and basement membrane (BM)23 (see Figure 1-16).
The extracellular matrix is secreted by fibroblasts (“fiber formers”), local cells that are present in the matrix. The matrix and the cells within it are known collectively as connective tissue because they connect cells together to form tissue and organs. Human connective tissues are enormously varied. They can be hard and dense, like bone; flexible, like tendons or the dermis of the skin; resilient and shock-absorbing, like cartilage; or soft and transparent, like the jelly-like substance that fills the eye. In all these examples, the majority of the tissue is composed of extracellular matrix, and the cells that produce the matrix are scattered within it like raisins in a pudding24 (see Figure 1-16).
Specialized Cell Junctions
Cells in direct physical contact with neighboring cells are often linked together at specialized regions of their plasma membranes called cell junctions. Cell junctions have two main functions: (1) to hold cells together and (2) to allow small molecules to pass from cell to cell, allowing coordination of the activities of cells that form tissues. The three main types of cell junctions are (1) desmosomes (adhering junctions, or macula adherens), (2) tight junctions (impermeable junctions, or zonula occludens), and (3) gap junctions (adhering [communicating] junctions) (Figure 1-17, A). Together they form the junctional complex. Desmosomes hold cells together by forming either continuous bands or belts of epithelial sheets or button-like points of contact. Desmosomes also act as a system of braces to maintain structural stability. Tight junctions are barriers to diffusion, prevent the movement of substances through transport proteins in the plasma membrane, and prevent the leakage of small molecules between the plasma membranes of adjacent cells. Gap junctions are clusters of communicating tunnels, connexons, that allow small ions and molecules to pass directly from the inside of one cell to the inside of another. Connexons are joining proteins that extend outward from each of the adjacent plasma membranes (see Figure 1-17, C). The integrity of connexons and dysregulation of intercellular ion movement has been linked to certain disorders, for example, atrial fibrillation.25 Cells connected by gap junctions are considered ionically (electrically) and metabolically coupled. Gap junctions coordinate the activities of adjacent cells. They are important, for example, in synchronizing contractions of heart muscle cells through ionic coupling and in permitting action potentials to spread rapidly from cell to cell in neural tissues. The reason that gap junctions occur in tissues that are not electrically active is unknown. Although most gap junctions are associated with junctional complexes, they sometimes exist as independent structures.
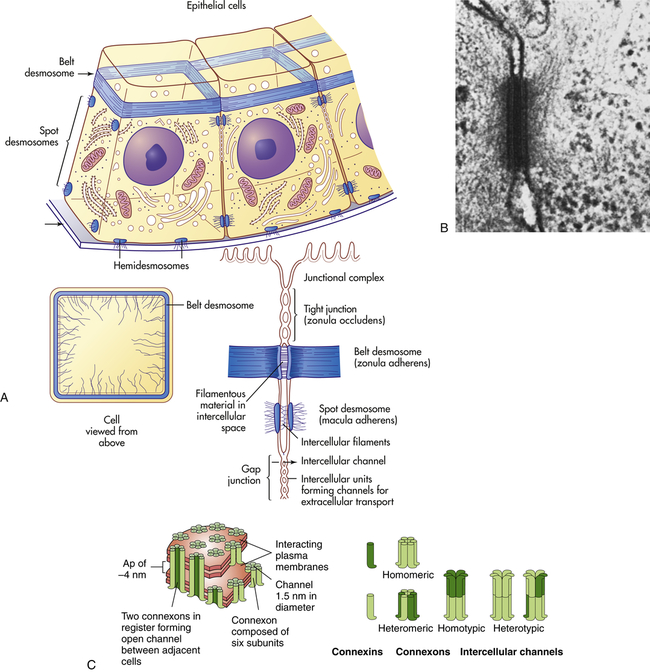
A, Schematic drawing of a belt desmosome between epithelial cells. This junction, also called zonula adherens, encircles each interacting cell. The spot desmosomes and hemidesmosomes, like the belt desmosomes, are adhering junctions. This tight junction is an impermeable junction that holds cells together but seals them in such a way that molecules cannot leak between them. The gap junction, as a communicating junction, mediates the passage of small molecules from one interacting cell to the other. B, Electron micrograph of desmosomes. C, Connexons. (A, B from Raven PH, Johnson GB: Biology, St Louis, 1992, Mosby. C from Alberts B et al: Molecular biology of the cell, ed 5, New York, 2008, Garland.)
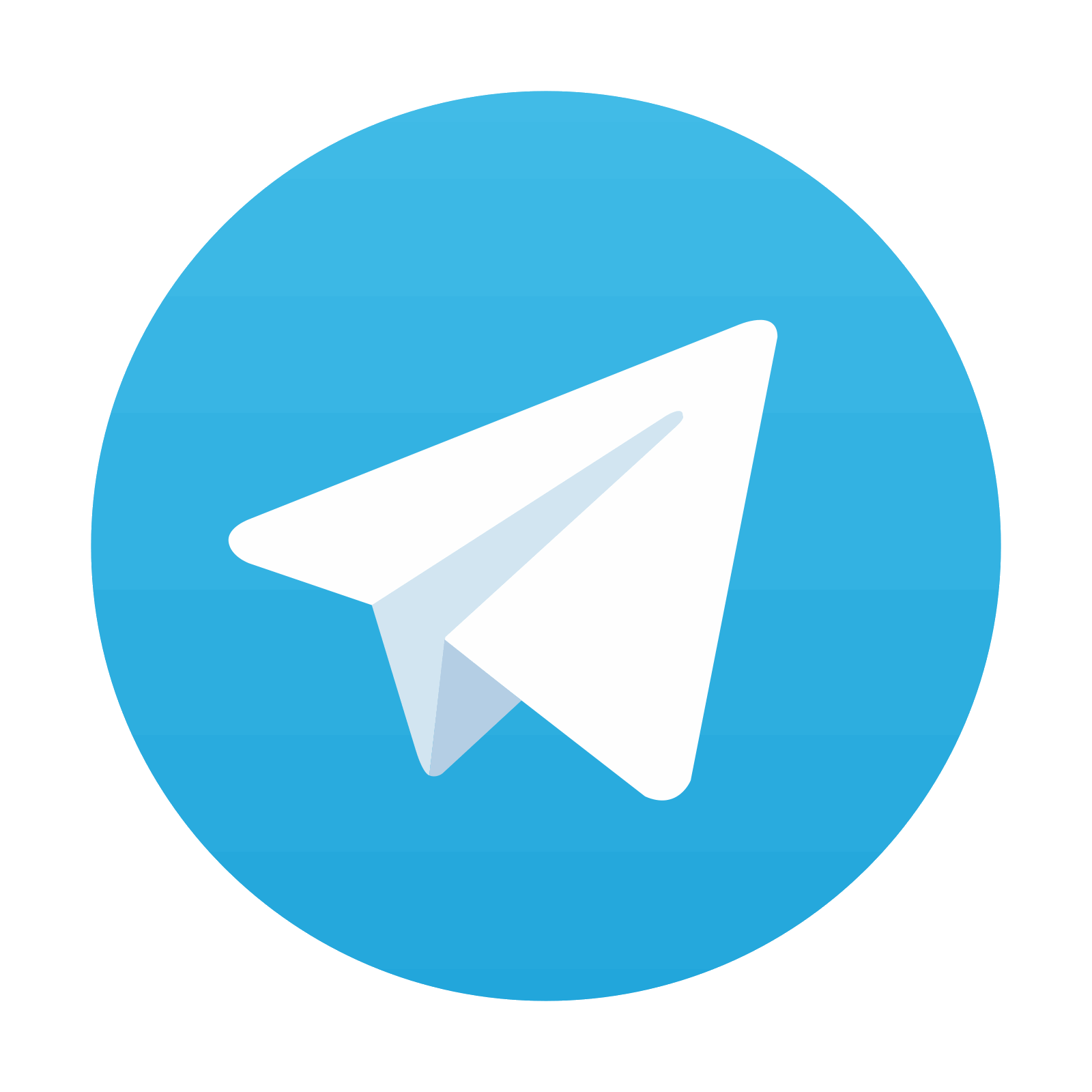
Stay updated, free articles. Join our Telegram channel
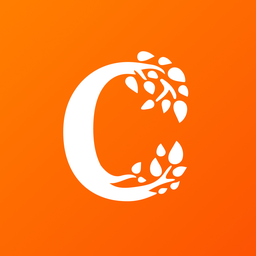
Full access? Get Clinical Tree
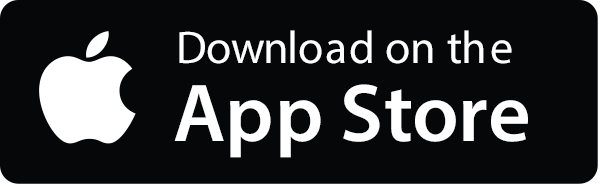
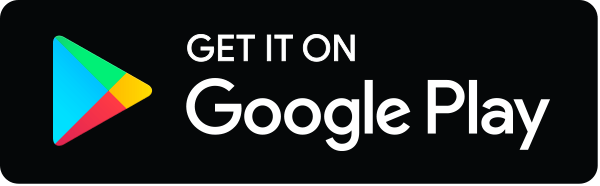