Carbohydrates present in food provide an average of 50% (range of about 32% to 70%) of the energy in the American diet, and the recommended intake range, or Acceptable Macronutrient Distribution Range (AMDR), is 45% to 65% of calories (Institute of Medicine [IOM], 2002). Carbohydrates, consumed as disaccharides, oligosaccharides, and polysaccharides, are digested, absorbed, and transported through the body primarily as glucose, although fructose and galactose are present as well. Glucose is the primary metabolic fuel in humans in the postprandial state due to the abundance of carbohydrate in the diet, the capacity of all tissues to catabolize it, and the limited capacity for glucose storage in the body. Tissue-specific differences in the pathways of glucose oxidation, glucose storage, glucose biosynthesis, and the utilization of glucose for the synthesis of other biomolecules are summarized in Figure 12-1 for liver, muscle, brain, and red blood cells. Pathways in the various tissues are coordinated to meet the metabolic challenges of the whole body. GLUT2 is a low-affinity glucose transporter that is localized primarily in the liver and the beta cells of the pancreas. To a lesser extent, it is found in the small intestine and kidneys. GLUT2 has a high Km for glucose and is a key transport protein involved in responding to elevated blood glucose in humans. In liver, because of the expression of GLUT2, maximal glucose uptake occurs when blood glucose levels are high, such as after a carbohydrate-containing meal. In the pancreatic beta cell, glucose uptake via GLUT2 signals that the blood glucose concentration has increased and begins the process by which the beta cell responds to elevated blood glucose with secretion of insulin into the bloodstream. In the pancreas, glucose is transported into the beta cell where it is rapidly phosphorylated to glucose 6-phosphate by a high Km glucokinase. The increase in glycolysis and oxidative metabolism of glucose increases the ATP/ADP ratio leading to a series of intracellular changes that result in the fusion of insulin-containing storage vesicles with the cell membrane and the release of insulin to the bloodstream (Figure 12-2). The combined action of GLUT2 and glucokinase allows the pancreatic beta cell to “sense” the blood glucose concentration and respond by increased secretion of insulin (Postic et al., 2001). In the small intestine and kidney tubules, GLUT2 is involved in glucose absorption and reabsorption, respectively, across the basolateral membranes of the epithelial cell barriers of these two tissues. Expression of the gene for GLUT2, SLC2A2, is regulated in a tissue-specific manner. In liver, SLC2A2 expression does not appear to be regulated by dietary carbohydrate or insulin, and GLUT2 levels in liver have not been found to change consistently in response to altered metabolic states. However, SCL2A2 gene expression in pancreatic beta cells is decreased when blood glucose levels are low. Conversely, a rise in blood glucose levels increases SCL2A2 messenger RNA (mRNA) and protein levels in pancreatic beta cells. SCL2A2 knockout mice are unable to sense glucose concentrations in the blood and exhibit impaired insulin secretion. This was rescued by reexpression of the SCL2A2 gene in pancreatic cells, which restored insulin secretion by the pancreas (Thorens, 2002). GLUT4 is present primarily in skeletal and cardiac muscle and in white and brown adipose tissue and is the only insulin-responsive glucose transporter. GLUT4 is encoded by the SLC2A4 gene. GLUT4 has a unique physiological role in whole-body glucose homeostasis. In effect, GLUT4 mediates the second-tier response to elevated blood glucose. In skeletal and cardiac muscle and adipose tissue, insulin stimulates a rapid translocation of preformed GLUT4 from intracellular vesicles in the cytosol to the plasma membrane of the cell surface (Czech, 1995). By increasing the concentration of glucose transporters at the cell surface, insulin increases the capacity for glucose uptake. Insulin signaling is known to activate AS160 (Akt substrate of 160 kDa), which is a Rab GTPase-activating protein, and TC10, which is a GTP-binding protein, both of which are required for insulin-stimulated GLUT4 translocation (Miinea et al., 2005). When insulin levels fall, GLUT4 is returned to the intracellular vesicular system by endocytosis. In unstimulated muscle and adipose cells, approximately 90% of GLUT4 is intracellular (Ishiki and Klip, 2005). Upregulation of GLUT4 translocation is disrupted in individuals with insulin resistance due to accumulation of intramyocellular lipid that interferes with insulin signaling (Petersen and Shulman, 2006). Exercise can also stimulate GLUT4 translocation to the plasma membrane in skeletal muscle, but this involves a different signaling pathway than that initiated by insulin (Uldry and Thorens, 2004). Exercise induces the cumulative effects of numerous inputs, including adenosine monophosphate (AMP)-activated protein kinase (AMPK) and increased cytosolic calcium (Ca2+) levels as major factors. There may be distal convergence between the insulin and exercise signaling pathways regulating GLUT4 translocation in skeletal muscle (Cartee and Funai, 2009). In addition to affecting the translocation of existing GLUT4 molecules to the plasma membrane, fasting, refeeding, and insulin level can also affect the expression of new GLUT4 molecules. Fasting or type 2 diabetes leads to a significant decrease in SLC2A4 mRNA and GLUT4 protein in adipocytes. This decrease can be reversed by carbohydrate refeeding or insulin treatment. A depletion in SLC2A4 mRNA and protein results in a decrease in the vesicular GLUT4 pool that is available for translocation to the plasma membrane. The decrease in SLC2A4 gene expression and the decrease in insulin-stimulated GLUT4 translocation both contribute to the decrease in glucose uptake in adipocytes with insulin deprivation (Figure 12-3). Expression of the SLC2A4 gene has also been investigated in skeletal muscle. Because the red and white muscle fiber types have different insulin sensitivities and GLUT4 concentrations, it has been difficult to determine the hormonal responsiveness of the SLC2A4 gene in skeletal muscle. Most studies indicate that SLC2A4 expression in muscle is not decreased in patients with insulin resistance/type 2 diabetes. This is surprising because skeletal muscle is responsible for at least 50% of glucose uptake from the blood after a carbohydrate-containing meal, whereas adipose tissue is responsible for much less. Studies in mice in which the SLC2A4 gene was selectively ablated in specific tissues, such as skeletal muscle and adipose tissue, indicated that there is “cross talk” between these two tissues, such that a decrease in adipose GLUT4 level (and therefore glucose uptake) decreases glucose uptake in skeletal muscle (Minokoshi et al., 2003). Exceptions to facilitated glucose uptake occur in the brush border membrane of the small intestinal absorptive cells and the renal proximal tubules, where glucose is taken up into the epithelial cells by an active symport process mediated by the sodium/glucose cotransporter (SGLT1), which is encoded by the gene SLC5A1. In this active symport, glucose is moved into the cell by a transport protein that first binds Na+. The energy for glucose uptake is provided by the Na+,K+-ATPase, which maintains a low intracellular Na+ concentration. Glucose, after entry into the epithelial cell, dissociates from the transport protein. (See Chapters 7 and 8 for more discussion of glucose transport in the intestine.) The series of enzymatic reactions that make up the glycolytic pathway are subdivided into two stages, as shown in Figure 12-4: (1) the priming of glucose, which requires ATP expenditure to generate phosphorylated intermediates; and (2) the production of reducing equivalents and the synthesis of ATP. The enzymes that catalyze the reactions of glycolysis are present in the cell cytosol, organized into multienzyme complexes that function together to channel the intermediates from one enzyme to another so that they do not become diluted in the cytosol. The glycolytic enzymes are associated with cellular structures such as actin filaments, microtubules, or the outer membranes of mitochondria (Lehninger et al., 1993). It should be noted that glucose 6-phosphate from glycogen does not require the hexokinase reaction. In skeletal muscle, glucose 6-phosphate from glycogen breakdown is a major substrate for glycolysis. Glucokinase (hexokinase IV), on the other hand, is the major hexokinase isoform in liver parenchymal cells and in the pancreatic beta cells. In contrast to hexokinase I, glucokinase has a low affinity for glucose, with a Km of approximately 10 mM. In addition, glucokinase is not inhibited by glucose 6-phosphate. The activity of glucokinase is linked to that of the high Km glucose transporter GLUT2, which is the major glucose carrier in liver cells and pancreatic beta cells. Because GLUT2 and glucokinase are not saturated at physiological glucose concentrations and because glucokinase is not inhibited by its product glucose 6-phosphate, they are able to respond to marked changes in blood glucose concentration. Thus GLUT2 and glucokinase in liver and the pancreatic beta cells allow uptake of glucose in proportion to its plasma concentration and the rapid conversion of this glucose to glucose 6-phosphate when blood glucose concentrations are elevated (e.g., after a carbohydrate-containing meal). Hepatocytes and pancreatic beta cells differentially regulate expression of the glucokinase gene by use of different promoters, different transcription start sites, and alternative splicing to generate glucokinase isoforms that have different N-terminal sequences (Magnuson and Jetton, 1993; Postic et al., 2001). Use of different gene promoters allows regulation of liver glucokinase in response to insulin and glucagon while pancreatic glucokinase is regulated in response to blood glucose concentration. This allows the liver to maintain blood glucose levels in the normal physiological range and the pancreas to sense elevations in blood glucose concentrations and respond with insulin secretion In this reaction, there is an intramolecular shift of a hydrogen atom, changing the location of the double bond. Phosphoglucose isomerase functions close to equilibrium, and therefore the reaction is reversible under intracellular conditions. The 6-phosphofructo-1-kinase reaction utilizes a second molecule of ATP and is the first committed step in glycolysis. Unlike glucose 6-phosphate, fructose 1,6-bisphosphate cannot be used directly as substrate for alternative pathways such as glycogen synthesis. The 6-phosphofructo-1-kinase reaction is highly regulated by allosteric modifiers and is one of the major determinants of the rate of glycolytic conversion of glucose to pyruvate. It is also regulated by changes in gene expression. In humans, there are three isoenzymes of 6-phosphofructo-1-kinase, each encoded by a separate gene. Hepatic mRNA levels of the 6-phosphofructo-1-kinase gene (PFK) are increased in response to carbohydrate refeeding after a fast (Granner and Pilkis, 1990), but in skeletal muscle there are no changes in expression of the PFK gene with either diabetes, insulin treatment, or exercise (Vestergaard, 1999). Glyceraldehyde 3-phosphate is in the direct path of glycolysis, but dihydroxyacetone phosphate is not. Dihydroxyacetone phosphate is isomerized to glyceraldehyde 3-phosphate via the action of triose-phosphate isomerase. The net result of the aldolase and triose-phosphate isomerase reactions is the production of two molecules of glyceraldehyde 3-phosphate. The series of reactions that converts one molecule of glucose to two molecules of glyceraldehyde 3-phosphate constitutes the first phase of glycolysis in which the chemical energy of ATP is used to generate phosphorylated intermediates. The dihydroxyacetone phosphate produced by the aldolase reaction may also be reduced to glycerol 3-phosphate and used for synthesis of glycerolipids; this is a particularly important source of glycerol 3-phosphate for triacylglycerol synthesis in small intestinal enterocytes and in adipocytes. This reaction incorporates inorganic phosphate (Pi) to produce a high-energy phosphate bond in 1,3-bisphosphoglycerate. The second product of the reaction, NADH, provides reducing equivalents for the energy conversion of electron transport and production of ATP by oxidative phosphorylation. This is the only glycolytic reaction that generates reducing equivalents for electron transport. It is important to note that the coenzyme NAD+ is present in limited amounts in the cytosol. For this reason, the NAD+ used in the glyceraldehyde 3-phosphate reaction needs to be regenerated in the cytosolic compartment so that NAD+ availability does not limit glycolysis. The enzyme that catalyzes this reaction is phosphoglycerate kinase. This is the first reaction in glycolysis that generates ATP. The formation of ATP by transfer of the phosphate group from 1,3-bisphosphoglycerate to ADP is a substrate-level phosphorylation. This reaction is catalyzed by pyruvate kinase and is the second substrate-level phosphorylation step that occurs in glycolysis. The pyruvate kinase reaction is essentially irreversible under intracellular conditions. This enzyme is highly regulated by allosteric and covalent modification, much like 6-phosphofructose-1-kinase, as is discussed in subsequent text of this chapter. Pyruvate produced by glycolysis can be metabolized in different ways, depending upon the availability of oxygen and the metabolic state of the cell. Pyruvate may enter the mitochondrion where it is converted to acetyl-CoA in a complex series of reactions catalyzed by the multienzyme pyruvate dehydrogenase complex. Acetyl-CoA so produced can enter the citric acid cycle for complete oxidation to CO2 and H2O, or it can exit the mitochondria as citrate and be used for fatty acid or sterol synthesis in certain cell types (see Chapter 16 for a discussion of de novo lipogenesis from glucose). In the absence of sufficient oxygen or in cells lacking mitochondria, pyruvate has a different fate; it is reduced to lactate by the cytosolic enzyme lactate dehydrogenase. In the presence of sufficient molecular oxygen and in cells with mitochondria, reducing equivalents (NADH + H+) produced by the glyceraldehyde 3-phosphate dehydrogenase reaction can be shuttled to the mitochondria by the reduction of metabolic intermediates in the cytosol, regenerating NAD+ in this compartment. The reduced intermediate is shuttled across the inner mitochondrial membrane with subsequent oxidation, thereby regenerating NADH + H+ from NAD+ in the mitochondria. NADH in the mitochondria is an electron donor, transferring reducing equivalents to Complex I (NADH dehydrogenase complex) in the series of oxidation–reduction reactions of electron transport (see Chapter 21, Figure 21-4). Under these conditions, the reduction of pyruvate to lactate is not required for the regeneration of NAD+ in the cytosol.
Carbohydrate Metabolism
Synthesis and Oxidation
Overview of Tissue-Specific Glucose Metabolism
Transport of Glucose Across Cell Membranes
Facilitated Transport of Glucose: the GLUT Transporters
GLUT2
GLUT4
Active Uptake of Glucose: Sodium-Dependent Glucose Transport
Glycolysis
Initial Steps of Glycolysis: The Priming of Glucose
Generation of Reducing Equivalents and Substrate-Level Synthesis of ATP
Further Metabolism of Pyruvate
Carbohydrate Metabolism: Synthesis and Oxidation
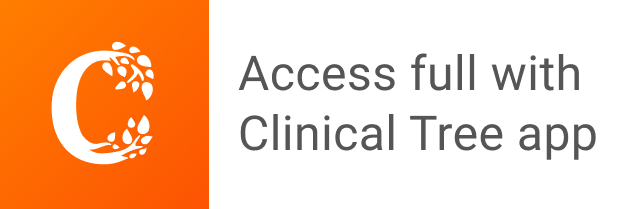