A. UNDERLYING PRINCIPLES
Figure 3.1 shows the three main reaction manifolds that underlie materials synthesis. The main sources of synthesized materials are oil and minerals. As a general rule, organic compounds are obtained from oil rather than from elemental carbon. The initial steps in the synthesis of the organic compounds in oil were probably carried out by living organisms during the early history of the planet. Note, however, that considerable long-term interest lies in the use of carbon (coke) or methane as a starting point for the production of organic chemicals in preparation for the time when oil is no longer plentiful. The starting materials for the synthesis of inorganic compounds come from two sources—either directly from mineral oxides, carbonates, silicates, sulfides, sulfates, chlorides, and other compounds, or synthesized from the element rather than from a mineralogical precursor. An example of the first route is the preparation of glass and other ceramics by the reaction of silica (sand) with alkali carbonates or various oxides. For the second route, a mineral must first be reduced to the element, and the element is then used either directly as a useful material (metals, silicon) or as the starting point for further synthesis.
A third access route to materials is from plants or animals. Wood, cotton, leather, and wool are still materials of widespread importance despite the wide choice of synthetic materials.
An important principle in materials chemistry is that organic reactions are relatively slow and are controlled mainly by kinetics. The products obtained depend on time, temperature, and concentration. This is because many of the covalent bonds in organic compounds have a low polarity, and they are broken only with difficulty by polar reagents. For example, a compound such as chloroform, CHCl3, or chloro-benzene is stable to water at normal temperatures and is not hydrolyzed to an alcohol or phenol. The slowness of many organic reactions is the reason why the mechanisms of organic reactions may be studied fairly readily because reaction intermediates can be identified or isolated, and the process may be interrupted if necessary to maximize the yield of an intermediate product.
Figure 3.1. Starting points and pathways for materials synthesis.

On the other hand, the bonds in inorganic compounds tend to be more polar. They react rapidly with polar or ionic reagents. Thus, phosphorus trichloride or silicon tetrachloride, which have polar P–Cl or Si—Cl bonds, are hydrolyzed almost instantaneously to the transient species P(OH)3 or Si(OH)4 when brought into contact with moisture. The coordination chemistry of inorganic compounds also tends to follow very rapid reaction kinetics. Because these types of inorganic reactions are so fast, the final products may be determined by thermodynamics rather than kinetics. Often an inorganic reaction will go to completion and will establish an equilibrium concentration of products before the investigator has time to study the pathways followed.
B. STARTING POINTS FOR MATERIALS SYNTHESIS—ISOLATION OF ELEMENTS
The fields of organic and inorganic reaction chemistry are widely diversified, and the following observations introduce a few principles that are significant in materials research. The abbreviated summary given here is designed to serve as a reminder of ideas from elementary inorganic and organic chemistry and to serve as a starting point for further reading. Note that inorganic, organic, and organometallic chemistry are now very closely related fields, and that many reaction methods, mechanisms, and techniques are common to all three.
The elements that are most widely used in materials synthesis are isolated from minerals (especially oxides or sulfides) by a process of reduction. Reduction is accomplished in one of several ways, which are summarized in reactions 1–9:
a. Reaction of an Oxide at High Temperatures with Carbon or Hydrogen.
The underlying principle is that carbon (usually in the form of coke) will react with a limited supply of oxygen to form carbon monoxide (reaction 1). Carbon monoxide is a powerful reducing agent that not only converts an oxide to the elemental form but also is itself oxidized to carbon dioxide. Alternatively, the carbon may react directly with the oxide to form carbon monoxide and dioxide.
Reduction by carbon is a widely used and inexpensive process that is employed for the isolation of iron and steel, chromium, silicon, phosphorus, tin, lead, copper, nickel, and numerous other elements. In some processes, heating is accomplished by the combustion of carbon. In others, heat is generated by striking an arc between two electrodes.
The carbon reduction process cannot be used effectively if the element reacts with carbon to form a stable carbide. For example, titanium forms a highly stable carbide, and this metal must be isolated by more complex procedures (see text below). Although iron reacts with carbon at high temperatures, the carbon content can be reduced by subsequent treatment of the molten metal with oxygen. Pure metals are seldom produced by carbon reduction, and subsequent purification steps are usually needed.
The use of hydrogen as a reducing agent is more expensive and requires more sophisticated equipment, but it can give pure material, often at lower temperatures. Thus, tungsten, which forms a stable carbide when heated with carbon, is obtained via the reduction of tungsten oxide (WO3) by hydrogen at 850°C (reaction 2). Germanium is isolated from GeO2 by reduction with hydrogen at temperatures above 500°C.
b. Reaction of an Oxide with an Element that Has a Greater Affinity for Oxygen.
Boron is isolated by the high-temperature reaction of boric oxide (B2O3) with magnesium (reaction 3). Magnesium is obtained from dolomite [MgCa(CO3)2] by heating with ferrosilicon at 1150°C (reaction 4).
c. Isolation via Chlorination or Oxidation.
Titanium is a difficult metal to extract from its ores (which explains its high cost). The ore, rutile (TiO2) or ilmenite (FeTiO3), is first heated with carbon and chlorine to produce either TiCl4, or TiCl4 plus FeCl3, which are separated by fractional distillation (reaction 5). The high-temperature interaction of TiCl4 with magnesium or sodium then yields titanium metal contaminated with MgCl2 or NaCl. These salts must then be extracted by treatment with aqueous acid to leave a titanium sponge. Titanium has a melting point of 1667°C, and is thus difficult to fabricate.
Copper is separated from its sulfide ores and from iron sulfide by a complex oxidation–reduction process. In this, the FeS is first oxidized by air to FeO and some of the Cu2S forms Cu2O. The Cu2O then reacts with unchanged Cu2S to form impure copper metal and SO2 (reaction 6). The loss of gaseous SO2 is a driving force for the reaction. Copper produced in this way is not pure enough for electrical applications, and it must be purified further by electrolysis.
d. Electrolytic Reduction.
Electrolysis is an excellent method for the production of high-purity metals. The penalty that must be paid is the high cost of electricity. Hence, electrorefining plants are normally situated close to supplies of relatively inexpensive hydroelectricity. Metals such as copper, magnesium, and aluminum are produced on a very large scale by electrolysis (reactions 7–9). In the case of magnesium, molten anhydrous MgCl2 or hydrated MgCl2 from seawater is electrolyzed at temperatures up to 750°C. Aluminum is produced from the mineral bauxite [Al(O)OH or Al(OH)3], which is first converted to Al2O3 and then electrolyzed at 940–980°C in molten cryolite, Na3AlF6 (reaction 8).
Impure copper or nickel, produced by the carbon reduction process, is cast into anodes and is electrolyzed using an electrolyte of aqueous copper sulfate or nickel sulfate. High-purity copper is deposited on the cathode. Silver, an impurity in the copper, deposits beneath the anode during the electrorefining process. The isolation of specific metals is discussed in Chapter 8.
e. Pyrolysis and Vapor Deposition.
An important access route to elemental carbon is via the pyrolysis of methane or other hydrocarbons to give carbon black or activated carbon. A similar material is soot, formed by vapor deposition in chimneys. Fullerenes and nanotubes are also produced by pyrolysis and vapor deposition. Diamond-like films are deposited on surfaces when gaseous methane or other hydrocarbons in the presence of hydrogen are heated by a plasma or by a wire at 2200°C and the products are allowed to impinge on the surfaces of metals or silicon. This chemical vapor deposition (CVD) of carbon onto metal surfaces yields thin, ultrahard films that are valuable for machine tools (Figure 3.2). Note that synthetic diamonds (i.e., discrete crystals) are produced from molten graphite by the application of heat (1500°C) and high pressure [58,000 atmospheres (atm)].
Figure 3.2. Chemical vapor deposition method for depositing diamond-like carbon on a substrate.

Other sources of elemental carbon are organic polymers, pitch, or asphalt, which, when pyrolyzed in the absence of oxygen, yield carbon fiber, which is an increasingly important material in aerospace applications (see Chapter 7). Finally, one of the major sources of elemental carbon is coke—a porous residue left after the volatile components have been distilled from coal. Coke is one of the least expensive reducing agents known, and its use in steelmaking revolutionalized industrial practice during the industrial revolution.
Another example of pyrolytic chemical vapor deposition is the conversion of silane, SiH4, to films of amorphous semiconductor silicon. This is a method for the preparation of relatively inexpensive semiconductors for solar cells (see Chapter 10).
Note that some relatively pure elements are found native in mineral deposits—gold, silver, sulfur, diamond, and graphite are well-known examples.
C. PRINCIPLES THAT UNDERLIE MATERIALS SYNTHESIS
1. Importance of Halides in Materials Synthesis
The formation of chloro compounds is a key step in the conversion of nonmetallic elements to materials. This is typically accomplished by the direct reaction of the element or its oxide or of an organic compound with chlorine at elevated temperatures, as illustrated in reactions 10–13. Typical examples are the production of the chlorides of boron, silicon, aluminum, or phosphorus. However, conversion of most metals to their chlorides is more easily accomplished by reaction with hydrochloric acid (reaction 14). Organic chloro-compounds are produced not from elemental carbon but by the chlorination of hydrocarbons (reactions 15 and 16). Chlorination of methane gives CH3Cl, CH2Cl2, CHCl3, and CCl4. Chlorination of benzene gives mono-, di-, and trichlorobenzenes. Brominations proceed in a similar manner.
Chloro and bromo compounds play two crucial roles in the synthesis of materials and their precursors:
1. They are reagents for reactions with nucleophiles such as alkoxides, aryloxides, amines, and organometallic reagents to link organic units to inorganic ele ments or to organic substrates, as shown in in reactions 17–22.
2. Main-group inorganic halides can be hydrolyzed readily to hydroxyl derivatives (reactions 23–26). These, in turn, condense by elimination of water to form oxide ceramics.
2. Acidic Hydroxides and Condensation Reactions
Although the hydroxides of the common alkali and alkaline-earth elements (groups I and II) are well-known bases, the hydroxy derivatives of most of the non-metals and some metals are acids. Examples range from strong acids such as sulfuric O2S(OH)2, nitric O2NOH, or perchloric O3ClOH acids to the moderate or weak acids such as silicic Si(OH)4, phosphoric OP(OH)3, or boric B(OH)3 acid and the amphoteric (acidic or basic depending on conditions) aluminum hydroxide Al(OH)3. These species are acids because the oxygen atoms of the OH groups are linked to the central element through covalent bonds, whereas the hydrogen atom can readily dissociate from oxygen as a hydrogen cation.
The weaker polyhydroxy acids such as silicic, boric, or phosphoric acids play an important role in materials chemistry because they readily undergo condensation reactions to form polymers and ceramics. A condensation reaction involves the elimination of a small molecule (usually water) to bind two molecules together through an element–oxygen–element covalent linkage (reactions 27–29). If each participating molecule bears only one hydroxy group, the result is a dimer (reaction 27). Two hydroxy groups per molecule yield a cyclic molecule or a linear polymer (reaction 28), and three or four yield a crosslinked ultrastructure (reaction 29).
The functionality of a polyhydroxy monomer may be controlled by replacement of some of the hydroxyl groups by inactive organic units such as CH3 or C6H5 units or conversion to a salt linkage such as O− Na+. Thus, the functionality of silicic acid can be changed from Si(OH)4 to Si(CH3)(OH)3, Si(CH3)2(OH)2, or Si(CH3)3OH, or of phosphoric acid from OP(OH)3 to OP(ONa)(OH)2, or OP(ONa)2OH (reaction 30).
A significant difference between the condensation products of the weak hydroxy acids and those of the strong acids is the stability of the condensation products. Sulfuric acid loses water to form poly(sulfur trioxide) (reaction 31), but this macromolecule is instantly hydrolyzed back to sulfuric acid when exposed to even trace amounts of water. This is not the case for the condensation products from the weak acids, which tend to be much more stable in neutral aqueous media and hydrolyze only in strong acid or base.
Organic compounds that bear hydroxyl units also participate in condensation reactions. This is true irrespective of whether the OH unit is part of a carboxylic acid structure or the functional component of an alcohol. An example is the formation of a polyester as shown in reaction 32.
Water is the molecule that is normally eliminated in materials-related condensation reactions, but the loss of ammonia or other small molecules is also possible. A characteristic of condensation reactions is that they are often controlled by equilibria rather than kinetics. This means that in a closed system the (forward) condensation reaction is in equilibrium with the reverse process in which the newly formed bond is hydrolyzed and the two original separate molecules are re-formed (reaction 33).
However, removal of the water as the reaction proceeds retards the back reaction and ensures that the condensation process goes to completion. Hence, moderate to high temperatures are usually needed to ensure that a condensation process proceeds efficiently. Condensation reactions are the basis of the synthesis of silicates, titanates, polyphosphates, polyesters such as Dacron or Mylar, polyamides such as nylons or Kevlar, polyimides, melamine resins, and many more.
3. Metathetical Exchange Reactions
The term metathetical exchange refers to a process whereby two molecules or ionic species exchange similar units. An example is when an aqueous solution of potassium bromide is added to a solution of sodium chloride (reaction 34).
Either cation can interact with either anion. In fact, all four ions are dispersed in water, and the concept of discrete pairing does not apply. However, if one of the exchanged ion salts is now less soluble than the starting materials, it will crystallize or precipitate from solution. Partial removal of the solvent by evaporation will yield a similar result.
A classical example is the addition of aqueous sodium sulfate to a solution of barium chloride (reaction 35). Barium sulfate is so insoluble that it precipitates immediately and drives the reaction to completion. Addition of an aqueous solution of silver nitrate to a solution of sodium chloride results in the immediate precipitation of silver chloride. Because reactions of this type are so rapid, they are either controlled by equilibria or they are driven to completion by the insolubility of one of the products.
Metathetical exchange processes occur with covalent molecules also. For example, triethylphosphate can be converted partially or completely to tripropylphosphate by treatment with sodium propoxide as shown in reaction 36. The main difference from the other examples is that these reactions are slow, and they fall into a category known as nucleophilic substitutions.
4. Nucleophilic Substitution
The replacement of one component of a covalent molecule by another is a vital part of both organic and inorganic chemistry. A general illustration of the process is shown in reaction 37.
A nucleophilic substitution occurs when an anion (nucleophile) attacks the more positively charged atom of a polar bond. We will call this attacked atom the central atom. The more negatively charged component of that bond departs and its place is taken by the incoming unit. There are two extremes of this type of reaction. In the first, the incoming anion attaches itself to the central atom to give a transient intermediate that bears a negative charge (reaction 38). This is called an SN2 reaction because it is a bimolecular process. In practice, the leaving anion may depart as the incoming anion approaches, in a synchronous process. Alternatively, the departing anion may leave before the incoming anion arrives (reaction 39)—a so-called SN1 reaction, because the key step is a unimolecular process. SN1 and SN2 reactions can be identified by their kinetic characteristics—that is, whether they follow first-order, pseudo-first-order, or second-order kinetics. The more polar the original E—X bond, the more likely it is that SN1 kinetics will prevail. A characteristic of SN2 processes is that they are strongly influenced by the size of both the incoming and outgoing anions. Because the transition state in an SN2 process is more crowded than the ground state (e.g., a pentacoordinate, trigonal bipyramidal silicon or phosphorus atom), steric factors exert a strong influence on the energy barrier of the transition state, the speed of the reaction, and the nature of the products. Moreover, because the approach of the incoming anion is likely to be from a direction furthest removed from that of the departing anion, an SN2 reaction would be expected to lead to an inversion of configuration.
By contrast, because the leaving anion departs first in an SN1 reaction (reaction 39), retention or scrambling of the original configuration is to be expected, and the steric size of the incoming unit is less crucial. These factors are important for understanding the nature of the products from these reactions and for predicting the course of new reactions.
5. Electrophilic Substitution
Electrophilic reactions occur when a cation attacks a compound and displaces another positively charged species, often a proton (reactions 40 and 41). These reactions are common in organic chemistry.
For example, the nitration of toluene by a mixture of nitric and sulfuric acids proceeds by an electrophilic mechanism (reaction 40), as does the sulfonation of benzene (reaction 41). Chlorination and bromination of organic compounds involves electrophilic replacement of hydrogen by the halogen. An important ramification of electrophilic chemistry is the polymerization of olefins by carbonium ions, as illustrated in the initiation process shown in reaction 42. The carbonium ion formed in this reaction can then attack another olefin molecule and initiate a chain growth process. These reactions are discussed in more detail in Chapter 6.
6. Coordination Chemistry
Molecules that have excess valence shell electrons in one or more lone-pair orbitals can donate those electrons into the unfilled valence shells of acceptor molecules. This concept was introduced in Chapter 2. Two classical examples are the coordination compounds formed between ammonia and boranes and between phosphines and transition metals (reactions 43 and 44).
The synthesis of coordination compounds is normally a matter of bringing the donor and acceptor molecules together in a solvent that does not itself interfere with the process. Donor solvents such as ethers or amines are normally avoided. Crystallization or precipitation of the donor–acceptor complex then yields the solid material. Complex formation from the vapor state is also possible.
7. Branching and Crosslinking
Organic and inorganic polymer chains can be linear or branched. Branched macro-molecules take several different forms as shown in Figure 3.3. Branched structures entangle more easily in the solid or liquid states. They also inhibit crystallite formation. Branching usually occurs during an inorganic or organic polymer synthesis process when tri- or tetrafunctional monomers are used along with difunctional species. Branched molecules will dissolve in suitable solvents, although the rate of dissolution might be slow.
Crosslinks are covalent or ionic connections that are formed between polymer molecules or that join rings together to form ultrastructure materials (Figure 3.3). Rubber is a useful material only because it contains crosslinked chains that prevent the polymer molecules from sliding past each other when the material is stretched. Many inorganic materials such as silicates are crosslinked through Si—O–Si linkages formed via the condensation of Si—OH groups. It is even possible to understand the properties of diamond or silicon in terms of crosslinking between every atom in the crystal. A characteristic of crosslinked species is that they are insoluble in all solvents. Light crosslinking will give a material that absorbs a solvent and swells but that will not dissolve. Heavily crosslinked materials do not even swell in potential solvents. Three methods for forming crosslinks in organic macromole-cules are shown in reactions 45–47. Reaction 45 is a common outcome for organic materials and is driven by free-radical reactions. The process shown as reaction 46 is known as a “2 plus 2 cycloaddition,” and is induced by ultraviolet radiation. Ionic crosslinks exist when chains are linked through acid side groups connected to a divalent or trivalent cation such as Ca2+ or Al3+ (reaction 47). This is a common situation in inorganic materials. Ionic crosslinks of this type can often be disrupted by replacement of the multivalent cations by monovalent cations such as Na+. Cross-linking by condensation of hydroxyl groups is a common process in silicate chemistry.
Figure 3.3. Different polymer molecular architectures, each of which generates different properties. (From Allcock, H. R., Chemistry and Applications of Polyphosphazenes , Wiley-Interscience, Hoboken, NJ, 2003, p. 5.)

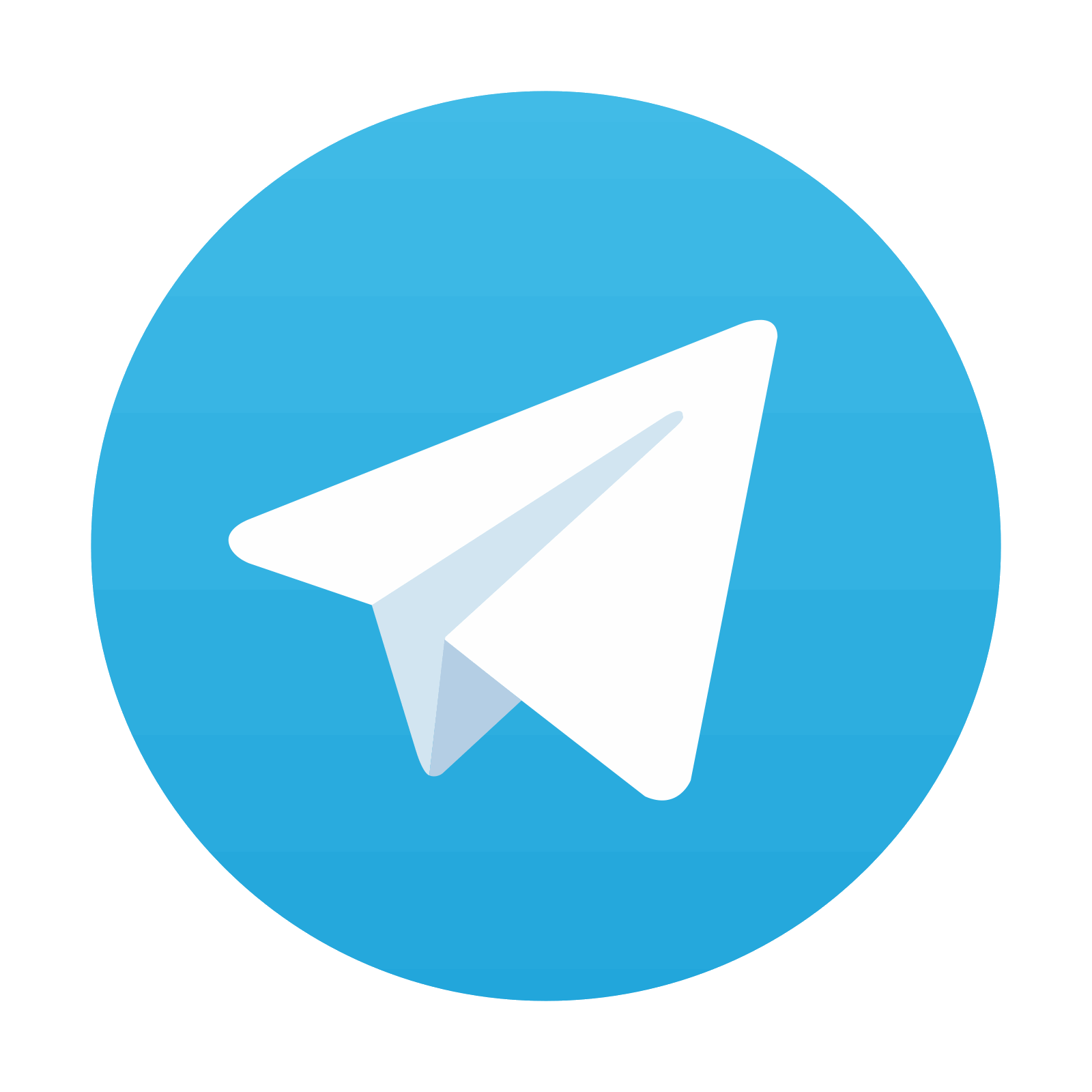
Stay updated, free articles. Join our Telegram channel
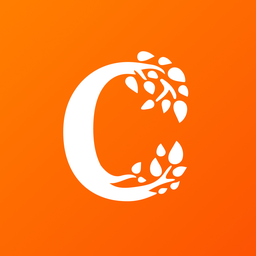
Full access? Get Clinical Tree
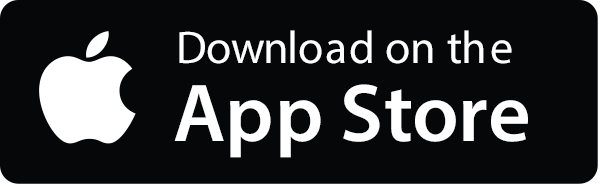
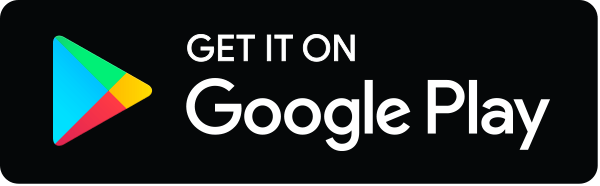