Chapter 19 Many small laboratories now have consolidated into larger, more efficient entities in response to market trends involving cost reduction. The drive to automate these mega-laboratories has led to new avenues in laboratory automation. No longer is automation simply being used to assist the laboratory technologist in test performance; it now includes (1) processing and transport of specimens, (2) loading of specimens into automated analyzers, (3) assessment of the results of the tests performed, and (4) storage of specimens. We believe that automating these additional functions is crucial to the future prosperity of the clinical laboratory.1,2 The following individual steps required to complete an analysis often are referred to collectively as unit operations (Box 19-1). These operations are described individually in this section, and examples demonstrate how they have been automated in terms of operational and analytical performance. In most automated systems, these steps usually are performed sequentially, but with some instruments, they may occur in parallel. Typically, the identifying link (identifier) between patient and specimen is noted at the patient’s bedside, and maintenance of this connection throughout (1) transport of the specimen to the laboratory, (2) subsequent specimen analysis, and (3) preparation of a report is essential. Several technologies are available for automatic identification and data collection purposes (Box 19-2). In practice, automatic identification includes only those technologies that electronically detect a unique characteristic or unique data string associated with a physical object. For example, identifiers such as (1) serial number, (2) part number, (3) color, (4) manufacturer, (5) patient name, and (6) medical record number have been used to identify an object or patient through the use of electronic data processing. In the clinical laboratory, labeling with a bar code has become the technology of choice for purposes of automatic identification. 1. Elimination of work lists for the system 2. Avoidance of mistakes made in the placement of tubes in the analyzer or during sampling 3. Avoidance of the need for analysis of specimens in a defined sequence Examples of types of bar codes that are used in chemistry analyzers are illustrated in Figure 19-1. When whole blood is used in an assay system, specimen preparation time is essentially eliminated. Automated or semiautomated ion-selective electrodes, which measure ion activity in whole blood rather than ion concentration, have been incorporated into automated systems to provide certain test results within minutes of the drawing of a specimen. This approach now is used commonly for assays of electrolytes and some other common analytes. Another approach involves manual or automated application of whole blood to dry reagent films and visual or instrumental observation of a quantitative change (see Chapter 20). Automated guided vehicles (AGVs), also called mobile robots, have been used successfully to transport laboratory specimens both within the laboratory and outside the central laboratory.13 They are easily adapted to carry various sizes and shapes of specimen containers and are reprogrammable with changes in laboratory geometry. In addition, in a busy laboratory setting, delivery of specimens to laboratory benches by a mobile robot can be more frequent than human pickup and has been shown to be cost-effective. Inexpensive models follow a line on the floor, whereas others have more sophisticated guidance systems. Their limitations include the need to batch specimens for greater efficiency and, in most cases, the requirement for laboratory personnel to place specimens onto or remove specimens from the mobile robot at each stopping place. Some mobile robots have been integrated with robotic systems that automate loading and unloading of specimens; others initiate an audible or visual signal of their arrival at a specified station so that employees are able to load or unload the specimens being transported. Box 19-3 lists several vendors that provide mobile robot systems for clinical laboratories. In most situations, the specimen for automatic analysis is serum or plasma. Many analyzers directly sample serum from primary collection tubes of various sizes. With such analyzers, the collection tubes most frequently used contain separator material that forms a barrier between serum or plasma and cells (see Chapter 7). Pipettes may be categorized as (1) fixed-, (2) variable-, or (3) selectable-volume (see Chapter 9). Selectable-volume pipettes allow the selection of a limited number of predetermined volumes. Pipettes with unlimited or selectable volumes are used in systems that allow many different applications, whereas fixed-volume pipettes usually are used for samples and reagents in instruments dedicated to the performance of only a small variety of tests.
Automation in the Clinical Laboratory
Automation of the Analytical Processes
Specimen Identification
Bar Coding
Specimen Preparation
Use of Whole Blood for Analysis
Specimen Delivery
Mobile Robots
Specimen Loading and Aspiration
Sample Introduction and Internal Transport
Discrete Processing Systems
Automation in the Clinical Laboratory
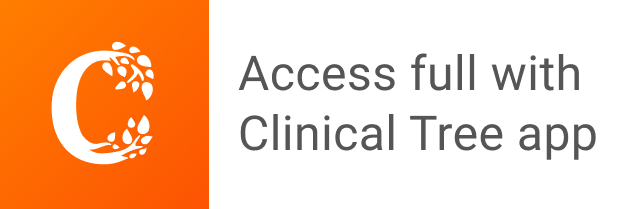