33 Attacking the enemy
antimicrobial agents and chemotherapy
The interactions between host, microbial pathogen and antimicrobial agent can be considered as a triangle, and any alteration in one side will inevitably affect the other two sides (Fig. 33.1). In this chapter, two sides of the triangle will be examined in greater detail:
• the interactions between antimicrobial agents and microorganisms
• the interactions between antimicrobial agents and the human host.
Selective toxicity
The term ‘selective toxicity’ was proposed by the immunochemist Paul Ehrlich (Box 33.1, Fig. 33.2). Selective toxicity is achieved by exploiting differences in the structure and metabolism of microorganisms and host cells; ideally, the antimicrobial agent should act at a target site present in the infecting organism, but absent from host cells. This is more likely to be achievable in microorganisms that are prokaryotes than in those that are eukaryotes, as the former are structurally more distinct from the host cells. (A comparison of the cellular organization of prokaryotic and eukaryotic cells is given in Ch. 1.) At the other end of the spectrum, viruses are difficult to attack because of their obligate intracellular lifestyle. A successful antiviral agent must be able to enter the host cell, but inhibit and damage only a virus-specific target. The desirable features of ideal antimicrobial agents are summarized in Box 33.2.
Box 33.1 Lessons in Microbiology
Paul Ehrlich (1854–1915)
Just as Pasteur towers over immunomicrobiology, Ehrlich (Fig. 33.2) is the father figure of immunochemistry. His contributions to the science of medicine at all levels are quite extraordinary. He was the first to propose that foreign antigens were recognized by ‘side-chains’ on cells (1890), a brilliant insight that took 70 years to confirm. He also discovered the mast cell, invented the acid-fast stain for the tubercle bacillus, and devised a method to manufacture and commercialize a strong diphtheria antitoxin. He pioneered the development of antibiotics with his work on ‘606’ (or ‘Salvarsan’), a treatment for syphilis, for which he was denounced by the church for interfering with God’s punishment for sin.
Box 33.2 Desired Properties of a New Antimicrobial Agent
a The desired attribute depends on drug usage. Narrow-spectrum drugs cause less disturbance to normal flora and may contribute less to emergence of antibiotic resistance, whereas broad-spectrum compounds are more useful for empiric therapy and treatment of polymicrobial infections. CSF, cerebrospinal fluid.
Discovery and design of antimicrobial agents
The discovery of new antimicrobial agents used to be entirely a matter of chance. Pharmaceutical companies undertook massive screening programmes searching for new soil microorganisms that produced antibiotic activity. In the light of our greater understanding of the mechanisms of action of existing antimicrobials, the processes have become rationalized, searching either for new natural products by target-site-directed screening or synthesizing molecules predicted to interact with a microbial target. Genomic approaches to the identification of novel targets have revolutionized this approach. In addition, knowledge of the crystal structure of the key enzymes involved in viral replication such as protease, reverse transcriptase and helicase leads to the design of new drugs. The steps in a rational design programme are summarized in Box 33.3.
Classification of antibacterial agents
There are three ways of classifying antibacterial agents:
There are five main target sites for antibacterial action
These targets differ to a greater or lesser degree from those in the host (human) cells and so allow inhibition of the bacterial cell without concomitant inhibition of the equivalent mammalian cell targets (selective toxicity).
Resistance to antibacterial agents
The genetics of resistance
In parallel with the rapid development of a wide range of antibacterial agents since the 1940s, bacteria have proved extremely adept at developing resistance to each new agent that comes along. This is illustrated for Staphylococcus aureus by the timeline shown in Figure 33.3. The rapidly increasing incidence of resistance associated with slowing down in the discovery of novel antibacterial agents to combat resistant strains is now recognized worldwide as a serious threat to the treatment of life-threatening infections.

Figure 33.3 ‘Time line’ illustrating the chronological emergence of antibiotic resistance in Gram-positive cocci.
Chromosomal mutation may result in resistance to a class of antimicrobial agents (cross-resistance)
• a single chromosomal mutation in one bacterial cell resulting in the synthesis of an altered protein: for example, streptomycin resistance via alteration in a ribosomal protein, or the single amino acid change in the enzyme dihydropteroate synthetase resulting in a lowered affinity for sulphonamides. A mutational event could also alter (i.e., increase or decrease) the production of a protein resulting in increased resistance.
• a series of mutations, for example changes in penicillin-binding proteins (PBPs) in penicillin-resistant pneumococci.
In the presence of antibiotic, these spontaneous mutants have a selective advantage to survive and outgrow the susceptible population (Fig. 33.4A). They can also spread to other sites in the same patient or by cross-infection to other patients and therefore become disseminated. Chromosomal mutations are relatively rare events (i.e. usually found once in a population of 106–108 organisms) and generally provide resistance to a single class of antimicrobials (i.e. ‘cross-resistance’ to structurally related compounds).
Genes on transmissible plasmids may result in resistance to different classes of antimicrobial agents (multiple resistance)
Not content with surviving the antibacterial onslaught by relying on random chromosomal mutation, bacteria are also able to acquire resistance genes on transmissible plasmids (Fig. 33.4B; see also Ch. 2). Such plasmids often code for resistance determinants to several unrelated families of antibacterial agents. Therefore a cell may acquire ‘multiple’ resistance to many different drugs (i.e. in different classes) at once, a process much more efficient than chromosomal mutation. This so-called ‘infectious resistance’ was first described by Japanese workers studying enteric bacteria, but is now recognized to be widespread throughout the bacterial world. Some plasmids are promiscuous, crossing species barriers, and the same resistance gene is therefore found in widely different species. For example, TEM-1, the most common plasmid-mediated beta-lactamase in Gram-negative bacteria, is widespread in E. coli and other enterobacteria and also accounts for penicillin resistance in Neisseria gonorrhoeae and ampicillin resistance in H. influenzae.
Resistance may be acquired from transposons and other mobile elements
Resistance genes may also occur on transposons; the so-called ‘jumping genes’, which by a replicative process are capable of generating copies which may integrate into the chromosome or into plasmids (see Ch. 2). The chromosome provides a more stable location for the genes, but they will be disseminated only as rapidly as the bacteria divide. Transposon copies moving from the chromosome to plasmids are disseminated more rapidly. Transposition can also occur between plasmids, for example, from a non-transmissible to a transmissible plasmid, again accelerating dissemination (Fig. 33.4C).
‘Cassettes’ of resistance genes may be organized into genetic elements called integrons
As discussed previously, antibiotic-resistance genes may individually reside on plasmids, the chromosome, or on transposons found in both locations. However, in some instances multiple resistance genes may come together in a structure known as an integron. As shown in Figure 33.5A, the integron encodes a site-specific recombination enzyme (int gene; integrase), which allows insertion (and also excision) of antibiotic-resistance gene ‘cassettes’ (resistance gene plus additional sequences including an ‘attachment’ region) into the integron attachment site (att). In classic operon fashion, a strong integron promoter controls transcription of the inserted genes. Based on their integration mechanism (integrase, etc.), integrons have been organized into different classes found in both Gram-negative and Gram-positive organisms. Whether acting as independent mobile genetic elements or inserted into transposons, integrons are capable of moving into a variety of DNA molecules, the overall hierarchy of which is depicted in Figure 33.5B. With their ability to capture, organize and rearrange different antibiotic-resistance genes, integrons represent an important mechanism for the spread of multiple antibiotic resistance in clinically important microorganisms.
Mechanisms of resistance
Resistance mechanisms can be broadly classified into three main types. These are summarized below, in Table 33.1 and described in more detail where relevant for each antibiotic in later parts of this chapter. Where bacterial mechanisms of antimicrobial resistance have been elucidated, they appear to involve the synthesis of new or altered proteins. As mentioned above, the genes encoding these proteins may be found on plasmids or the chromosome.
Access to the target site may be altered (altered uptake or increased exit)
This mechanism involves decreasing the amount of drug that reaches the target by either:
Classes of antibacterial agents
The following parts of this chapter deal with groups of antibacterial agents based on their target site and chemical structure. In each case, the discussion attempts to summarize the answers to the questions set out in Table 33.2, reviewing the interactions between antibacterial agent and bacteria and between the antibacterial and the host (i.e. two sides of the triangle in Fig. 33.1).
Table 33.2 In order to understand the nature and optimum use of an antibacterial agent, the questions listed here must be answered
What is it? | Chemical structure: natural or synthetic product |
What does it do? | Target site, mechanism of action |
Where does it go? (and therefore preferred route of administration) | Absorption, distribution, metabolism and excretion of the drug in the body of the host |
When is it used? | Spectrum of activity and important clinical uses |
What are the limitations to its use? | Toxicity to the human host; lack of toxicity, i.e. resistance of the bacteria |
How much does it cost? | Great variation between agents but cost is a serious limitation on availability of some agents in resource-poor countries |
Inhibitors of cell wall synthesis
Peptidoglycan, a vital component of the bacterial cell wall (see Ch. 2), is a compound unique to bacteria and therefore provides an optimum target for selective toxicity. Synthesis of peptidoglycan precursors starts in the cytoplasm; wall subunits are then transported across the cytoplasmic membrane and finally inserted into the growing peptidoglycan molecule. Several different stages are therefore potential targets for inhibition (Fig. 33.6). The antibacterials that inhibit cell wall synthesis are varied in chemical structure. The most important of these agents are the beta-lactams, the largest group, and the glycopeptides which are active only against Gram-positive organisms. Bacitracin (primarily used topically) and cycloserine (mainly used as a ‘second-line’ medication for treatment of tuberculosis, discussed later in this chapter) have many fewer clinical applications.
Beta-lactams
Beta-lactams contain a beta-lactam ring and inhibit cell wall synthesis by binding to penicillin-binding proteins (PBPs)
Beta-lactams comprise a very large family of different groups of bactericidal compounds, all containing the beta-lactam ring. The different groups within the family are distinguished by the structure of the ring attached to the beta-lactam ring – in penicillins this is a five-membered ring, in cephalosporins a six-membered ring – and by the side chains attached to these rings (Fig. 33.7).
PBPs are membrane proteins (e.g. carboxypeptidases, transglycosylases and transpeptidases) capable of binding to penicillin (hence the name PBP) and are responsible for the final stages of cross-linking of the bacterial cell wall structure. Inhibition of one or more of these essential enzymes results in an accumulation of precursor cell wall units, leading to activation of the cell’s autolytic system and cell lysis (Fig. 33.8).
Different beta-lactams have different clinical uses, but are not active against species that lack a cell wall
A vast array of beta-lactam antibiotics are currently registered for clinical use. Some, such as penicillin, are active mainly against Gram-positive organisms, whereas others (e.g. semi-synthetic penicillins, carboxypenems, monobactams, second-, third- and fourth-generation cephalosporins) have been developed for their activity against Gram-negative rods. Only the more recent beta-lactams are active against innately more resistant organisms such as Pseudomonas aeruginosa (Table 33.3).
Table 33.3 Characteristics of representative beta-lactams
Drug class | Category | General spectrum of activity |
---|---|---|
Penicillins | ||
Penicillin G, Va | Natural penicillin | Gram-positive bacteria |
Cloxacillina Dicloxacillina Nafcillina Oxacillina | ![]() | ![]() |
Amoxicillina,b Ampicillina,b | ![]() | Gram-positive bacteria Gram-negative bacteria, including spirochetes, Listeria monocytogenes, Proteus mirabilis and some Escherichia coli |
Carbenicillina Ticarcillinb Mezlocillin Piperacillinb | Semisynthetic (carboxy) penicillin Semisynthetic (ureido) penicillin | Gram-positive bacteria Enhanced coverage of Gram-negatives, including Pseudomonas and Klebsiella |
Cephalosporins | ||
Cefadroxila Cefazolin Cephalexina Cephalothin Cephradinea | ![]() | ![]() |
Cefaclora Cefamandole Cefonicid Cefprozila Cefuroximea | ![]() | |
Cefdinira Cefditorena Cefoperazone Cefpodoximea Cefotaxime Ceftazidime Ceftibutena Ceftizoxime Ceftriaxone | ![]() | |
Cefepime Cefpirome | ![]() | Improved activity against Gram-negative bacteria |
Ceftaroline | Anti-MRSA | Improved activity, especially against MRSA |
Cephamycinc | ||
Cefmetazole Cefotetan Cefoxitin | Gram-positive bacteria Improved activity against Bacillus fragilis | |
Carbapenems | ||
Ertapenem Imipenem Meropenem Doripenem | Gram-positive and Gram-negative bacteria | |
Monobactams | ||
Aztreonam | Gram-negative bacteria including Haemophilus influenza and Pseudomonas aeruginosa |
Although there are many beta-lactam agents available, the most commonly used ones are listed, together with their main indications.
b Can be formulated in combination with beta-lactamase inhibitors (see Fig. 33.9).
c Often classified with second generation cephalospoxins.
Resistance to beta-lactams may involve one or more of the three possible mechanisms
Resistance by production of beta-lactamases
The beta-lactamases of Gram-positive bacteria are released into the extracellular environment (Fig. 33.8A) and resistance will only be manifest when a large population of cells is present. The beta-lactamases of Gram-negative cells, however, remain within the periplasm (Fig. 33.8B).
To date, hundreds of different beta-lactamase enzymes have been described. All have the same function but with differing amino acid sequences that influence their affinity for different beta-lactam substrates. Some enzymes specifically target penicillins or cephalosporins, while others are especially troublesome in broadly attacking most beta-lactam compounds (i.e. extended-spectrum beta-lactamases, ESBLs). Some beta-lactam antibiotics (e.g. carbapenems) are hydrolysed by very few enzymes (beta-lactamase stable), whereas others (e.g. ampicillin) are much more labile. Beta-lactamase inhibitors such as clavulanic acid (Fig. 33.9) are molecules that contain a beta-lactam ring and act as ‘suicide inhibitors’, binding to beta-lactamases and preventing them from destroying beta-lactams. They have little bactericidal activity of their own.
Glycopeptides
Glycopeptides are large molecules and act at an earlier stage than beta-lactams
Glycopeptides are bactericidal and interfere with cell wall synthesis by binding to terminal d-alanine-d-alanine at the end of pentapeptide chains that are part of the growing bacterial cell wall structure (see Fig. 33.6). This binding inhibits the transglycosylation reaction and prevents incorporation of new subunits into the growing cell wall. As glycopeptides act at an earlier stage than beta-lactams, it is not useful to combine glycopeptides and beta-lactams in the treatment of infections.
Both vancomycin and teicoplanin are active only against Gram-positive organisms
Vancomycin and teicoplanin are used mainly for:
• the treatment of infections caused by Gram-positive cocci and Gram-positive rods that are resistant to beta-lactam drugs, particularly multiresistant Staphylococcus aureus and Staphylococcus epidermidis
• for patients allergic to beta-lactams
• the treatment of Clostridium difficile in antibiotic-associated colitis, although concerns that this may promote emergence of glycopeptide-resistant enterococci in the gut flora have led to the increasing use of alternative compounds.
Resistance
Organisms may acquire resistance to glycopeptides
Historically, the most clinically relevant acquired glycopeptide resistance has been observed in Enterococcus faecium and Enterococcus faecalis (vancomycin-resistant enterococci; VRE), first reported by investigators in the UK in 1986. Since that time, a variety of resistance phenotypes have been described which can be differentiated by transferability (e.g. plasmid association), inducibility and extent of resistance (Table 33.4). The genes associated with the highest levels of glycopeptide resistance are vanA, vanB, and vanD which encode a ligase producing pentapeptides terminating in d-alanine-d-lactate.
Inhibitors of protein synthesis
Although protein synthesis proceeds in an essentially similar manner in prokaryotic and eukaryotic cells, it is possible to exploit the differences (e.g. 70 S vs 80 S ribosome) to achieve selective toxicity. The process of translation of the messenger RNA (mRNA) chain into its corresponding peptide chain is complex, and a range of antibacterial agents act as inhibitors, although the full details of their mechanisms of action are not yet known (Fig. 33.10).
Aminoglycosides
The aminoglycosides are a family of related molecules with bactericidal activity
The aminoglycosides contain either streptidine (streptomycin) or 2-deoxystreptamine (e.g. gentamicin; Table 33.5). The original structures have been modified chemically by changing the side chains to produce molecules such as amikacin and netilmicin that are active against organisms that have developed resistance to earlier aminoglycosides.
Table 33.5 Aminoglycoside-aminocyclitol antibiotics classified according to their chemical structure
4,6-distributed 2-deoxystreptamines | |
Gentamicina | Complex of 3 closely related structures; first aminoglycoside with broad spectrum |
Tobramycinb | Activity very similar to gentamicin but slightly better against Pseudomonas aeruginosa |
Amikacin | Semi-synthetic derivative of kanamycin; active against many gentamicin- resistant Gram-negative rods |
Netilmicinb | Activity spectrum similar to amikacin: possibly lower toxicity |
4,5-disubstituted 2-deoxystreptamines | |
Neomycinb | Too toxic for parenteral use but has topical uses in decontaminating mucosal surfaces |
Streptidine-containing | |
Streptomycinb | Oldest aminoglycoside; now use restricted to treatment of tuberculosis |
They are also differentiated by the genus of microorganisms that produces them, and this is reflected in the spelling of the names.
a Micins from Micromonospora species.
b Mycins from Streptomyces species.
Aminoglycosides act by binding to specific proteins in the 30 S ribosomal subunit, where they interfere with the binding of formylmethionyl-transfer RNA (fmet-tRNA) to the ribosome (Fig. 33.10), thereby preventing the formation of initiation complexes from which protein synthesis proceeds. In addition, aminoglycosides cause misreading of mRNA codons and tend to break apart functional polysomes (protein synthesis by multiple ribosomes tandemly attached to a single mRNA molecule) into non-functional monosomes.
Gentamicin and the newer aminoglycosides are used to treat serious Gram-negative infections
Gentamicin, tobramycin, amikacin and netilmicin are important for the treatment of serious Gram-negative infections, including those caused by P. aeruginosa (Box 33.4). They are not active against streptococci or anaerobes, but are active against staphylococci. Against P. aeruginosa, amikacin is most active. Amikacin and netilmicin may be active against strains resistant to gentamicin and tobramycin (see below). Streptomycin is now reserved almost entirely for the treatment of mycobacterial infections. Neomycin is not used for systemic treatment, but can be used orally in gut decontamination regimens in neutropenic patients.
Box 33.4 Indications for Aminoglycoside Therapy
Basic rule: use only in severe, life-threatening infections
• Gram-negative septicaemia (including Pseudomonas) usually in combination with beta-lactam
• Septicaemia of unknown aetiology arising from:a
• Bacterial endocarditis for synergy with beta-lactam
• Staphylococcus aureus septicaemia in combination with beta-lactam
• Pyelonephritis for difficult cases
• Post-surgical abdominal sepsis in combination with anti-anaerobe therapy.
Production of aminoglycoside-modifying enzymes is the principal cause of resistance to aminoglycosides
Production of aminoglycoside-modifying enzymes is the most important mechanism of acquired resistance (Fig. 33.11). The genes for these enzymes are often plasmid-mediated, located on transposons, and transferable from one bacterial species to another. The enzymes alter the structure of the aminoglycoside molecule, thus inactivating the drug. The type of enzyme determines the spectrum of resistance of the organism containing it.
Tetracyclines
Tetracyclines are bacteriostatic compounds that differ mainly in their pharmacological properties rather than in their antibacterial spectra
Tetracyclines are a family of large cyclic structures that have several sites for possible chemical substitutions (Fig. 33.12).
Tetracyclines inhibit protein synthesis by binding to the small ribosomal subunit in a manner that prevents aminoacyl transfer RNA from entering the acceptor sites on the ribosome (see Fig. 33.10). While this process may occur with both prokaryotic and eukaryotic ribosomes, the selective action of tetracyclines is due to their much greater uptake by prokaryotic cells.
Tetracyclines are active against a wide variety of bacteria, but their use is restricted due to widespread resistance
Tetracyclines are used in the treatment of infections caused by mycoplasmas, chlamydiae and rickettsiae. Resistance in other genera is common, due partly to the widespread use of these drugs in humans and also to their use as growth promoters in animal feed. The resistance genes are carried on a transposon, and new cytoplasmic membrane proteins are synthesized in the presence of tetracycline. As a result, tetracycline is positively pumped out of resistant cells (efflux mechanism). Although included with the tetracyclines (Fig. 33.12), tigecycline is a new member of a related class of compounds (glycylcyclines), derived from minocycline, with activity against bacteria resistant to tetracyclines.
Chloramphenicol
Chloramphenicol contains a nitrobenzene nucleus and prevents peptide bond synthesis, with a bacteriostatic result
Chloramphenicol has affinity for the large (50 S) ribosomal subunit where it blocks the action of peptidyl transferase, thereby preventing peptide bond synthesis (see Fig. 33.10). The drug has some inhibitory activity on human mitochondrial ribosomes (which are also 70 S) which may account for some of the dose-dependent toxicity to bone marrow (see below).
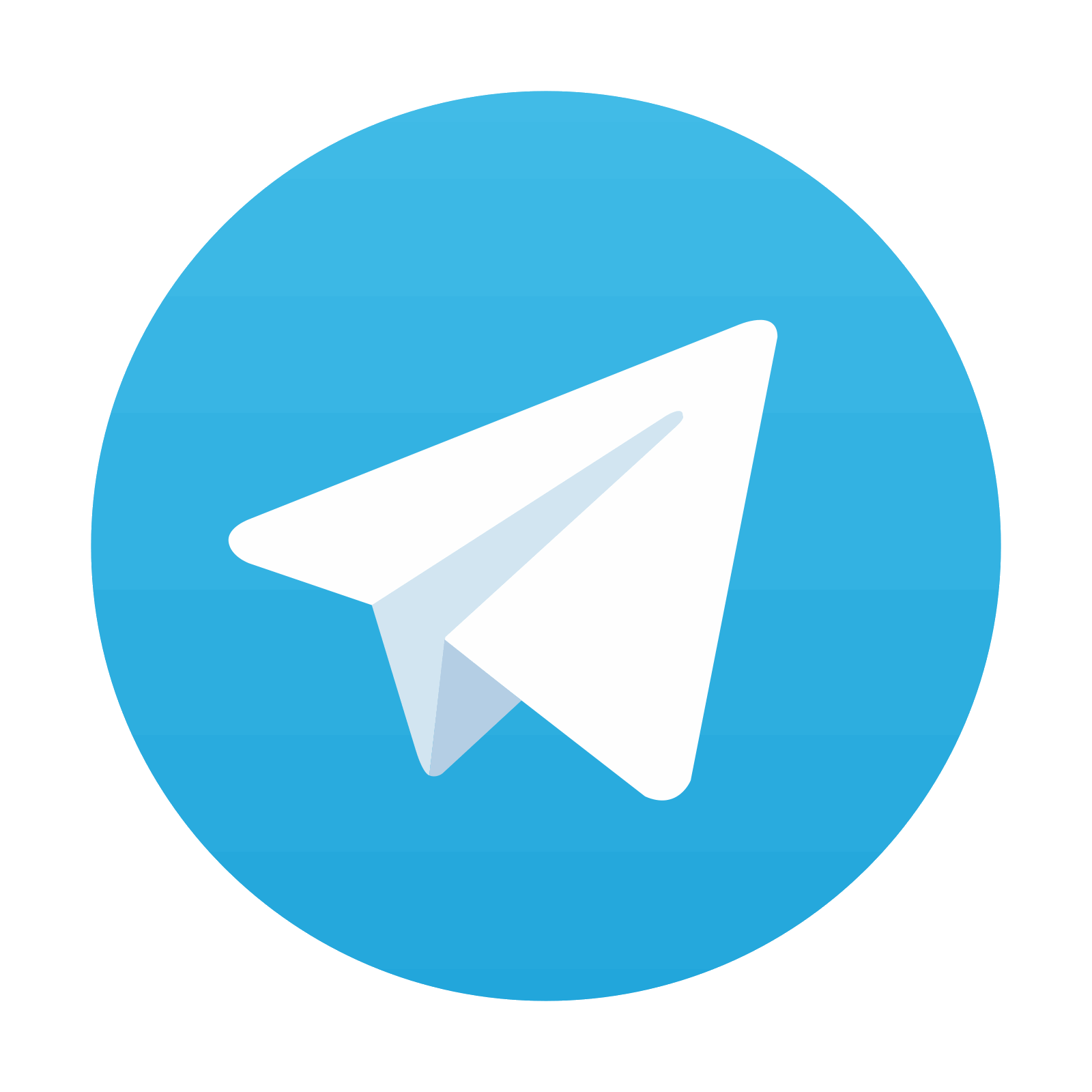
Stay updated, free articles. Join our Telegram channel
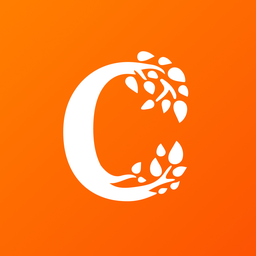
Full access? Get Clinical Tree
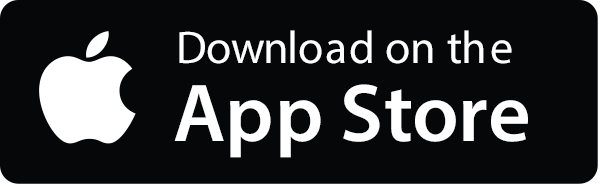
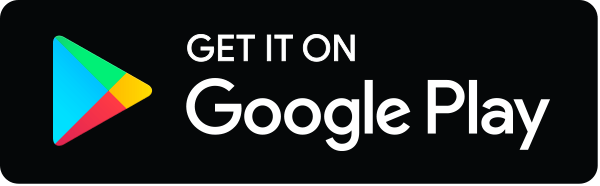