As with TB control, there have been numerous other advances in our understanding of M. tuberculosis. Perhaps the most notable advances emanated from the complete genome sequencing of multiple serovars of M. tuberculosis. Genome sequencing and other molecular genetic approaches led to a rapid increase in our knowledge of the genetic basis of drug resistance in M. tuberculosis including both identification of the target genes and the nature of the mutations that confer resistance (3). This led to a new generation of molecular techniques for rapid, direct detection of drug-resistant tubercle bacilli in clinical samples using nucleic acid amplification techniques (4). Two such methods, the Hain line probe assay (5) and the Cepheid GeneXpert (6) were emphatically endorsed by the WHO.
The Mycobacterium avium complex (MAC) was once a major cause of morbidity and mortality in patients with AIDS. However, with the implementation of highly active antiretroviral therapy and effective anti-MAC prophylaxis, disseminated MAC disease in HIV-positive patients has all but disappeared. In contrast, MAC continues to be a cause of difficult to treat pulmonary disease and appears to be more prevalent (7–9). Rapidly growing mycobacteria continue to be important causes of respiratory disease and disseminated cutaneous infections, although the antimicrobial armamentarium that is available for treating these infections has greatly improved (10). Phylogenetically, the rapidly growing mycobacteria are distinct and separate from the other mycobacteria. But, even within the slowly growing mycobacteria, there are considerable phylogenetic distinctions. These distinctions are reflected in the diverse relationships between mycobacterial species and a wide range in susceptibility and resistance to antimicrobial agents.
The authors of this chapter have strived to integrate conventional wisdom with new knowledge in an effort to provide reliable practical information about antimicrobial agents and mycobacteria for the use of both mycobacteriology specialists and clinicians.
IN VITRO SUSCEPTIBILITY TESTING METHODS
Mycobacterium tuberculosis Complex
M. tuberculosis is the most clinically important member of the M. tuberculosis complex, which includes M. tuberculosis, Mycobacterium bovis, M. bovis bacillus Calmette-Guérin (BCG), Mycobacterium africanum, Mycobacterium microti, and Mycobacterium caprae (11). The members of the complex are closely related based on DNA homology (12,13). Indeed, the degree of DNA homology indicates that the complex is more properly considered a collection of serovars or pathovars of the same species. Disease caused by M. bovis and M. africanum is clinically indistinguishable from disease caused by M. tuberculosis, and treatment is the same for all three “species” with the exception that M. bovis is inherently resistant to treatment with pyrazinamide (PZA). However, with pasteurization of milk, the isolation of M. bovis is uncommon and M. africanum is only rarely isolated from clinical specimens in the United States. M. microti causes a TB-like disease in voles and M. caprae is typically isolated from goats, but neither of these latter serovars is considered pathogenic for humans. Clearly, M. tuberculosis is the most common cause of both pulmonary and extrapulmonary TB in humans.
Treatment
The Centers for Disease Control and Prevention (CDC), the American Thoracic Society (ATS), and the Infectious Diseases Society of America (IDSA) in the United States now recommend the use of four drugs for the initial phase of treatment of M. tuberculosis pulmonary disease caused by drug-susceptible organisms that includes isoniazid, rifampin, ethambutol and PZA for two months of treatment (14). The initial phase should be followed by a “continuation phase” of 4 or 7 months of additional treatment with INH and rifampin or INH and rifapentine (15). Modifications of these regimens is dependent on a variety of factors, including HIV status, confirmation of drug susceptibility, and complicating factors such as young age, pregnancy, liver disease, and HIV infection (14). Recommendations are provided for appropriate modifications of the initial phase as well as the continuation phase of treatment, including when continuation treatment is appropriate (16).
Clearly, however, the treatment of pulmonary TB has become significantly more difficult because of the emergence of multiple drug resistance (MDR) and extensively drug resistance (XDR) as well as the large and growing numbers of TB patients coinfected with HIV.
The use of four drugs is the preferred regimen for compliant patients with a fully susceptible M. tuberculosis isolate. Ethambutol for adults or perhaps streptomycin for children who cannot be monitored for visual acuity should be included in the regimen until susceptibility results are known. At least two additional active agents should be added to failing treatment regimens in any patients with TB (1,17).
The early detection of drug resistance is essential for the successful management of MDRTB. In this regard, rifampin resistance is the most useful indicator of an MDR phenotype. A commercially available, highly automated real-time polymerase chain reaction (PCR) assay (Cepheid Xpert MTB/RIF) has been developed and has shown good performance in detection of rifampin resistance (18). Because the Xpert MTB/RIF assay is highly automated, it can be used in many settings, including laboratories where the level of training and expertise may not be sufficient to offer TB culture and drug susceptibility testing. This has led the WHO to strongly recommend that the “Xpert MTB/RIF be used as the initial diagnostic test in individuals suspected of MDRTB or HIV-associated TB” (6). Indeed, the XPERT MTB/RIF is likely to have broad application not only because of its ability to predict resistance to rifampin but also because it can simultaneously detect the presence of TB complex bacilli, including in HIV-infected patients and in children with a sensitivity that exceeds that of acid-fast microscopy for direct specimen testing (19–21). However, caution must be exercised in the interpretation of XPERT MTB/RIF results because this system also detects mutations in the rpoB gene that are not associated with rifampin resistance. These unassociated mutations can have a significant effect on predictive values in settings where the prevalence of rifampin resistance is not high (22).
Also, commercial PCR-based line probe assays have been developed which simultaneously detect the presence of M. tuberculosis complex and the presence or absence of mutations associated with first- or second-line drug resistance (23–25). These assays require greater technical expertise than the XPERT MTB/RIF assay but yield more information, including the ability to predict susceptibility or resistance to INH and some second-line drugs. For this reason, line probe assays also have been endorsed by WHO for use when drug-resistant TB is suspected, according to country-specific algorithms (5).
Real-time PCR assays such as XPERT MTB/RIF use molecular beacon probes to detect the presence or absence of wild-type nucleotide sequences as markers of rifampin susceptibility or resistance. One weakness of this approach is that not all polymorphisms within the target result in phenotypic rifampin resistance or may only cause small changes in quantitative drug susceptibility but the change may be sufficient to prevent hybridization to the reporter and the system will report such isolates as rifampin-resistant. Hain line probe assays incorporate DNA probes, which are directed against some of the most commonly occurring mutations associated with rifampin resistance. These mutant-specific probes improve the ability of the assay to specifically detect mutations associated with phenotypic resistance. However, inevitably, some mutations may be detected by probe-based systems, which are not associated with drug resistance. DNA sequencing systems, therefore, provides the most definitive detection of resistance compared with probe-based systems. Databases are already available and increasing in size, which associate particular gene sequences with drug susceptibility, resistance, and, in some cases, quantitative associations between nucleotide sequences and likely minimal inhibitory concentrations (MIC) (3).
Finally, the cost of DNA sequencing is rapidly decreasing, and applications have been developed for determining the nucleotide sequences of previously identified targets directly from clinical specimens (26–28).
Molecular versus Phenotypic Testing
Differences in genotypic (nucleotide sequence) and phenotypic (growth or inhibition of growth) test results are expected and observed. Where resources permit, it is sometimes best to use both genotypic and phenotypic testing to obtain the best prediction of drug efficacy. Among the differences that are known to result in such discrepancies are the following:
1. Not all mutations associated with drug resistance are known. For example, mutations in the katG gene and inhA promoter region account for most INH resistance, typically 80% to 90%, but not all (3,29,30).
2. Culture-based susceptibility testing will often use a class drug to predict susceptibility or resistance to several drugs within a class. For example, testing rifampin may serve to predict susceptibility to other rifamycins, and testing one fluoroquinolone drug may be used to predict susceptibility or resistance to all fluoroquinolones. However, specific mutations have been identified that genotypically distinguish rifampin-resistant versus rifabutin-susceptible isolates and ofloxacin-resistant versus moxifloxacin-susceptible isolates (31,32).
3. Mixed populations of drug-susceptible and drug-resistant bacilli may be simultaneously present as drug resistance develops. A molecular test may detect the predominant wild-type population at a point in time, whereas a growth-based test may reveal the emerging drug-resistant subpopulation of tubercle bacilli. Because resistant bacilli in a proportion of greater than 1% is associated with treatment failure, failure to detect minority populations of resistant bacilli can be considered a weakness of current molecular methods (33). However, in practical terms, given the selective presence of anti-TB drugs, the persistence of a mixed population of drug-susceptible and drug-resistant bacilli in a patient’s tissue is relatively brief, and correlations between phenotypic and genotypic results are high.
4. Molecular methods may reveal nucleotide polymorphisms of unknown significance within sequences previously associated with drug resistance. Some of these polymorphisms could lead to drug resistance even though the affected strain tests as susceptible in a growth-based test. For example, mutations in the rpoB gene have been described which lead to small MIC increases (34,35).
5. Finally, because susceptibility test results may not be reported for days to weeks depending on the availability of resources and location, patients with suspected MDRTB should be empirically treated with an injectable drug (e.g., amikacin or kanamycin), a fluoroquinolone, ethambutol, PZA, and perhaps a fifth drug (e.g., ethionamide, p-aminosalicylic acid [PAS], linezolid, clarithromycin, amoxicillin-clavulanate, rifabutin, or cycloserine) (36).
Despite the aforementioned limitations, there is increasing evidence that the sensitivity and specificity of molecular tests are in excess of 85% for several drugs. A compilation of sequence-based results in comparison with proportion susceptibility testing is shown in Table 5.1. For the first-line drugs, the sensitivities and specificities varied significantly depending on gene target, method, and perhaps operator.
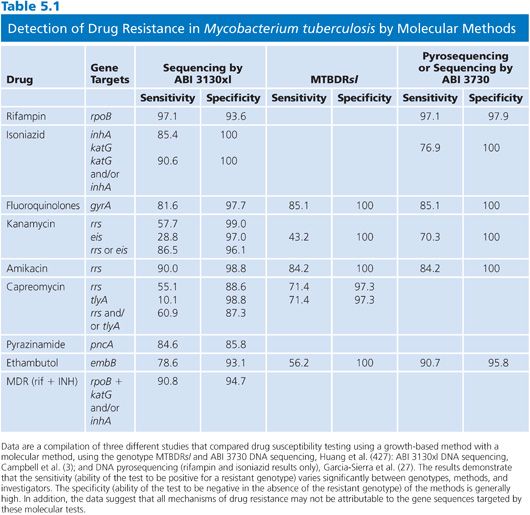
Drug Resistance and Critical Concentrations
An in vitro susceptibility test of M. tuberculosis is fundamentally a test to detect drug resistance, and the most reliable in vitro susceptibility test result that mycobacterial laboratories can report is that an isolate of M. tuberculosis is resistant to a drug (i.e., an isolate that tests susceptible to a drug may not be effectively treated for a variety of reasons, such as impaired drug uptake) because treatment with a drug to which M. tuberculosis is resistant invariably leads to therapeutic failure. Drug resistance is defined for M. tuberculosis in terms of the critical concentrations of drugs, and this concept is the basis for the most common M. tuberculosis drug susceptibility tests. David (37) showed in the early 1970s that the mutation frequencies for single-drug resistance to INH, rifampin, ethambutol, or streptomycin ranged from 1 × 10−7 to 2 × 10−10. The clinical significance of these numbers is clear when one considers that a single caseous lesion commonly found in pulmonary TB can contain 108 to 1010 tubercle bacilli. Thus, it is apparent why treatment of TB with a single agent invariably results in relapse due to a resistant isolate. Conversely, two or more agents prevent resistance because the frequency of multiple drug resistance is the product of the single-drug frequencies of mutation, that is, 10−14 to 10−17. If the frequency of resistant bacilli within a population is greater than 1% (usually much greater than 1%) in a previously untreated patient, this constitutes primary resistance. In most areas of the United States, for example, primary resistance to INH or streptomycin occurs in less than 7% of patients; however, in other areas of the world, primary resistance may occur in more than 50% of patients. If resistant bacilli are isolated from a patient in whom the initial isolates were susceptible, this constitutes secondary resistance; the transmission of these resistant organisms represents the most likely source of primary resistance in previously untreated patients.
The MDR phenotype develops as a result of the sequential accumulation of chromosomal mutations (38,39) and neither transposable elements nor plasmids have been associated with an MDR phenotype. In addition, the genetic basis for resistance is the same in MDR M. tuberculosis isolates from both HIV-positive and HIV-negative patients (38). No MDR genotypes have been described as being associated with a single genetic event or a single novel resistance determinant such as drug efflux.
Thus, the definition of critical concentrations is derived from two important observations. First, 95% or more of wild-type strains of M. tuberculosis are fully susceptible to first-line antimycobacterial agents, including INH, rifampin, ethambutol, PZA, and streptomycin, where a wild type refers to a strain of M. tuberculosis that has never been exposed to antimycobacterial agents. Second, the percentage of a population of tubercle bacilli that would make an isolate different from wild-type strains was defined by the WHO as ranging from 1% (INH and rifampin) to 10% (ethambutol and streptomycin) based, in part, on a correlation with therapeutic efficacy. For reasons of uniformity, 1% was adopted by the CDC as the threshold for all drugs tested in the United States. The critical concentration of a drug is then defined as the concentration of drug required to prevent growth above the 1% threshold of the test population of tubercle bacilli. The critical concentration for an antituberculous agent closely approximates the MIC for wild-type M. tuberculosis because the convention has been to define MICs in terms of an end point of 99% inhibition of growth (Table 5.1). Finally, it is important to understand that drugs are used in combination primarily to prevent the emergence of resistance. However, there is some evidence to indicate that first-line agents may act in a synergistic manner. This synergism may reflect combined effects on tubercle bacilli but most likely also reflects the fact that different drugs may act on tubercle bacilli in different physiologic states.
Special (Local) Populations Hypothesis
In considering the susceptibility of M. tuberculosis to antimicrobial agents, it is important to appreciate that the organism is likely to exist in the tissue under different physiologic conditions: (a) rapidly growing cells in the aerobic and neutral-pH environment of the pulmonary cavity; (b) slowly growing cells in the oxygen-depleted and low-pH environment of the caseous lesions, where the burden of tubercle bacilli is highest; (c) cells within macrophages; and (d) dormant tubercle bacilli, which are the most intractable to treatment and the likely source of reactivation. Mitchison (40) conceptualized this phenomenon as a special populations hypothesis, which posits that INH, rifampin, and streptomycin are most active against the relatively rapidly dividing bacilli; rifampin is likely to also be active against bacilli that grow in spurts, and PZA is active against bacilli in the acidic milieu of caseous lesions and in acidified vacuoles of macrophages. At present, there are no agents that are known to be active against dormant bacilli. Wayne and Sramek (41) provided laboratory evidence that metronidazole (a nitroimidazole) is active against dormant bacilli, but without therapeutic activity (42,43), but newer nitroimidazoles have promising activity (see the following texts).
Detection and Identification
The rapid and accurate detection and identification of M. tuberculosis not only is important for the diagnosis of disease but also for monitoring the response to therapy and for effective control of disease by public health authorities. In addition, the identification of M. tuberculosis is essential for accurate and reliable susceptibility testing; for example, the misidentification of M. avium as M. tuberculosis could result in a false report of MDR M. tuberculosis because M. avium is inherently resistant to INH and only variably susceptible to rifampin and ethambutol. Specimens submitted for culture include respiratory, urine, stool, sterile tissue (e.g., bone marrow), and blood samples. There are several commercial methods available for the detection of M. tuberculosis and other mycobacteria in clinical specimens including Septi-Chek (Becton Dickinson, Cockeysville, MD), Bactec MGIT 960 (Becton Dickinson, Sparks, MD), MB Redox (Heipha Diagnostica Biotest, Heidelberg, Germany), VersaTREK II (Trek Diagnostic Systems, Cleveland, OH), ALERT 3D (bioMerieux, Durham, NC), and Bactec 460 (Becton Dickinson, Heidelberg, Germany) (44).
The identification of several clinically significant mycobacteria can be achieved within a few hours using commercially available DNA probes (such as AccuProbe, Hologic/ Gen-Probe, Inc, San Diego, CA) that hybridize to species-specific ribosomal RNA (rRNA) sequences (45,46). AccuProbes have proven to be a reliable, fast, and cost-effective method for identifying certain specific mycobacteria isolated from clinical specimens. However, other rapid and reliable methods are available that potentially can identify a broader range of mycobacteria. These methods include transcription-mediated amplification, strand displacement amplification, 16S rDNA sequencing, multiplex PCRs, PCR restriction analysis, line probe assays high performance, liquid chromatography of mycolic acid derivatives, and matrix-assisted laser desorption/ionization time-of-flight mass spectroscopy (MALDI-TOF).
By combining a semiautomated method of detection and one of the newer methods of identification, a definitive laboratory diagnosis of mycobacterial infection should not take longer than 4 weeks. Indeed, it is not unreasonable for a clinician to expect reliable detection and (at least) presumptive identification of mycobacterial infection within 7 to 14 days. If it is not feasible to use a molecular or other rapid method for identification of mycobacteria, standard methods of identification can be improved by using a strategy that limits the number of biochemical tests (47).
SUSCEPTIBILITY TESTING
Methods and Standardization
There are four traditional methods for measuring the susceptibility of M. tuberculosis to the antimicrobial agents used for the treatment of TB: (a) agar proportion, (b) broth proportion, (c) absolute concentration, and (d) resistance ratio. Although there are advantages and disadvantages to each of these methods, there is some consensus that the proportion method is presently the most reliable test because the proportion procedure best controls for the inoculum size (48). Because the absolute concentration and resistance ratio methods are no longer widely used and not generally recommended, those methods are not discussed in detail.
The agar proportion method can be applied as either a direct or indirect test. In the direct test, a specimen that is smear-positive for acid-fast bacilli (AFB) is used as the source of inoculum for the susceptibility test and the specimen is inoculated directly onto the test media with and without drugs. In the indirect test, a pure culture, usually a subculture, of M. tuberculosis is used as the source of inoculum for the susceptibility test. On average, the results of the direct test are available 3 to 4 weeks before the results of an indirect test, using agar media.
Criteria for Performing Susceptibility Tests
The current recommendation is that all initial isolates of M. tuberculosis from a patient, regardless of the source of the specimen, should be tested and the results promptly reported to the health care provider and the health department/TB control (16). Beyond this, susceptibility tests should be performed on subsequent isolates if the patient’s cultures fail to convert to negative within 3 months or if there is clinical evidence of a failure to respond to therapy. Other indications for susceptibility testing include the following: (a) A patient produces specimens that contain an increased number of AFB after an initial decrease; (b) a patient is suspected to have primary resistance, that is, lives in an area with a high incidence of resistant TB or was exposed to resistant TB; or (c) the isolate is from a patient with meningitis or disseminated TB (49). The susceptibility testing of initial isolates is recommended for all patients, regardless of the (local) incidence of resistance. The testing of all initial isolates provides for the continuous surveillance of drug susceptibility patterns, which is important because these patterns provide the basis for initial empiric therapy. The issue of laboratory experience in susceptibility testing is controversial. Although the original recommendation that susceptibility tests be performed only in laboratories that are capable of species identification and that perform at least 10 susceptibility tests per week (50) was reasonable several years ago, most public health and many private laboratories test primary agents because of the reliability of commercial systems and the compelling need for a rapid turnaround time. Nevertheless, a laboratory that performs susceptibility tests should be able to identify the isolate as to species as a measure of competence. However, testing should be limited to first-line drugs, but the testing of second-line drugs should be referred to a qualified reference laboratory. Indeed, it is prudent to confirm resistance to first-line drugs, especially for initial isolates, by referring the isolate to another laboratory with more experience in susceptibility testing of mycobacteria.
Choice of Antimycobacterial Agents
Primary and secondary antimycobacterial agents are listed in Table 5.2, along with important pharmacokinetic information and the average MIC for susceptible strains of M. tuberculosis. Although the recommendation is to test PZA as a first-line agent, PZA testing remains somewhat problematic. If PZA is not routinely tested along with first-line agents, testing must be done as soon as there is evidence for resistance to the other first-line agents. While PZA monoresistant strains of M. tuberculosis are uncommon, the prevalence may be increasing (51). Second-line agents are usually tested only if an isolate is resistant to the primary agents or if the patient has failed therapy with first-line agents. An isolate of M. tuberculosis complex may be considered resistant to primary agents if it is resistant to rifampin or to any two first-line agents (49). Three different fluoroquinolones are commonly included as second-line agents (49). However, many laboratories do not test second-line agents and appropriately refer such requests to an experienced reference laboratory.
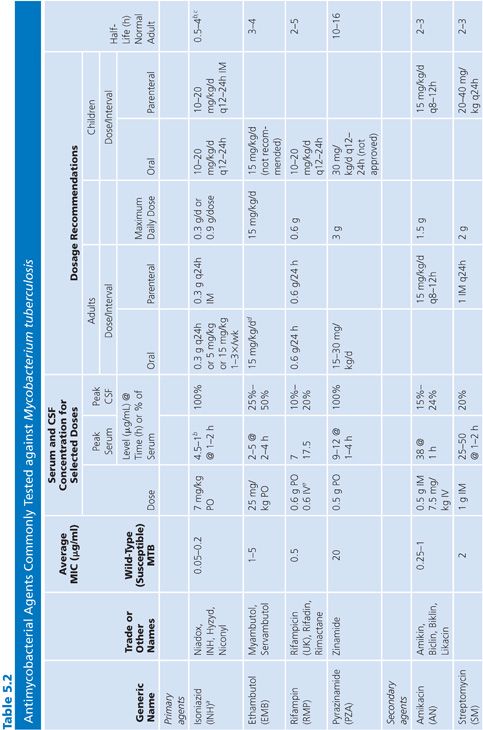
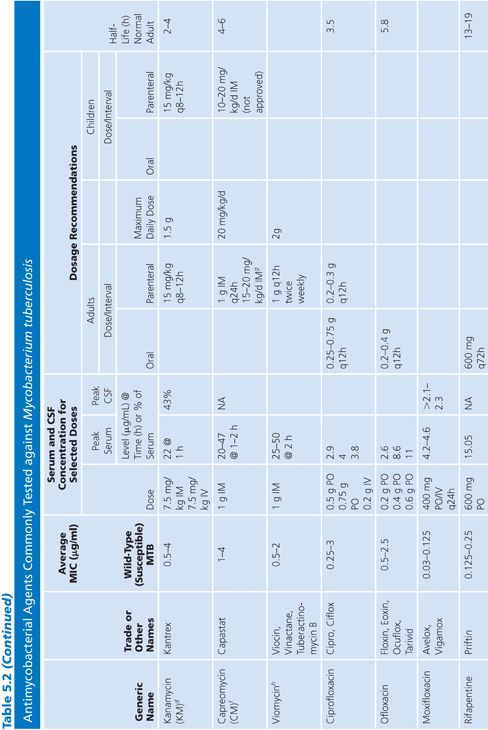
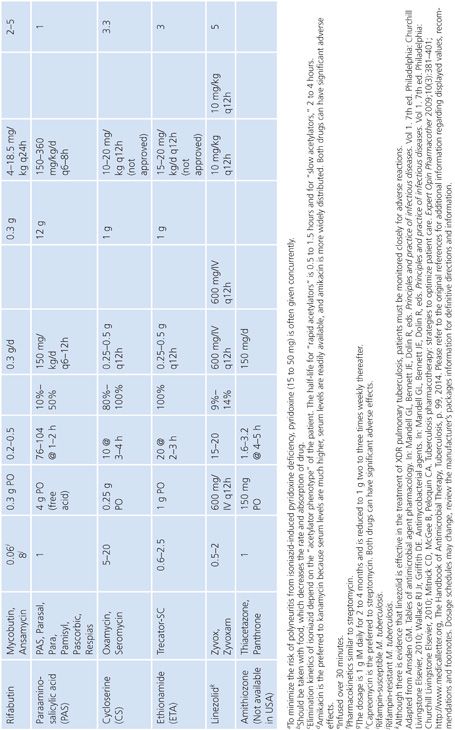
Sources of Antimycobacterial Agents
Antimicrobial reference powders can be obtained from commercial sources or from the manufacturer. In addition, most antimicrobial reference powders are available from the United States Pharmacopeial Convention (http://www.usp.org) or in Europe from the Zentrallaboratorium Deutscher Apotheker (http://www.zentrallabor.com). Antimicrobial agents formulated for therapeutic use in humans or animals should not be used for susceptibility testing. The potency (usually micrograms per milligram of powder) and expiration date must be known for each lot of drug, and the drugs should be stored as recommended by the manufacturer or, in the absence of recommendations, at −20°C in a desiccator under vacuum. The desiccator should be brought to room temperature before opening in order to avoid condensation and inadvertent hydration of the powders, which may affect the weight and activity of the drugs. The potency of a compound should take into account purity, water content, and active fraction (e.g., free base or acid vs. salt) (49).
Stock Solutions of Antimycobacterial Agents
Stock concentrations of drugs should be prepared on the basis of the potency and purity of the drug, which may vary from lot to lot. The required weight (using a fixed volume) or volume (using a fixed weight) for preparing a stock solution can be calculated using one of the following equations:
weight (mg) = [volume (mL) × concentration (μg/mL)]/potency (μg/mg)
volume (mL) = [weight (mg) × potency (μg/mg)]/concentration (μg/mL)
Stock solutions should be prepared at a concentration of at least 1,000 μg/mL, preferably 10,000 μg/mL or 10-fold higher than the highest concentration to be tested, whichever is greater. The drug should be dissolved in water or the smallest amount of solvent necessary to produce a clear solution. The solvent and diluent should be water, dimethyl sulfoxide, or buffer. In general, it is more accurate to carefully weigh a quantity of drug that is slightly in excess of the desired amount (50 to 100 mg) and adjust the volume of the solvent to achieve the desired final concentration. If necessary, the stock solution should be sterilized by aseptic filtration through a 0.22-μm pore membrane. Some drug solutions, such as rifampin, autosterilize. The stock solutions should be dispensed into screw-capped polypropylene tubes and stored at −70°C. Thawed tubes of stock drug solution should not be refrozen. Stock solutions prepared and stored in this manner have an expiration date of 1 year (or less) from the time of preparation or a length of time that is in accordance with the manufacturer’s recommendations (52). To add drug to media, a tube of the frozen stock solution is thawed and diluted with water or buffer to yield a solution of 100 to 10,000 μg/mL. The appropriate volume of diluted stock solution is added to 200 mL of sterile 7H10 medium to achieve the desired final concentration (Table 5.3).
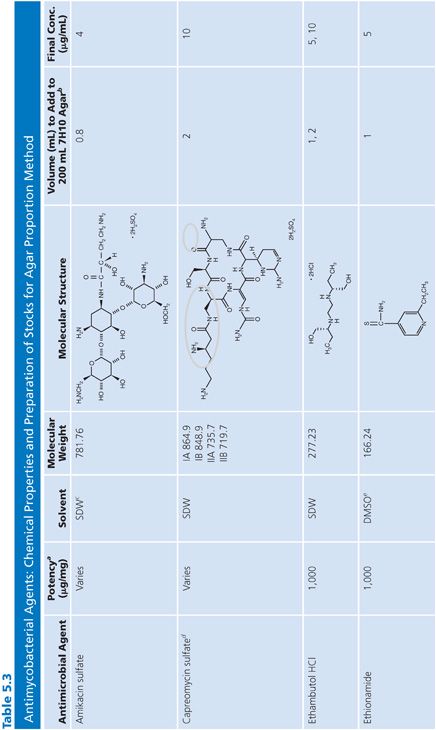
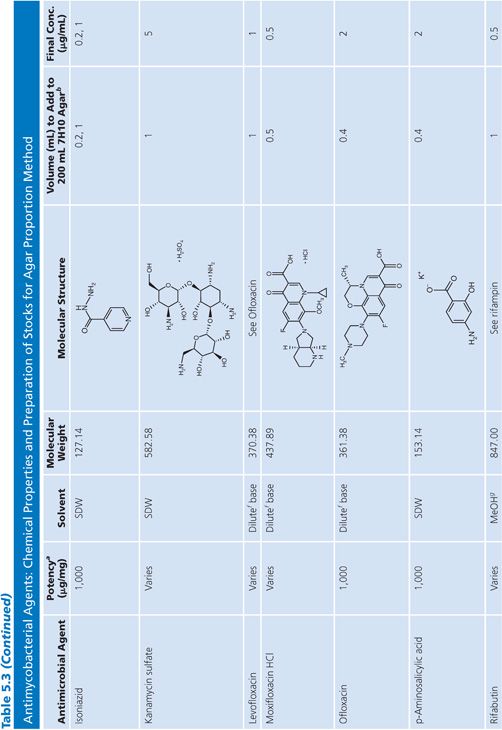
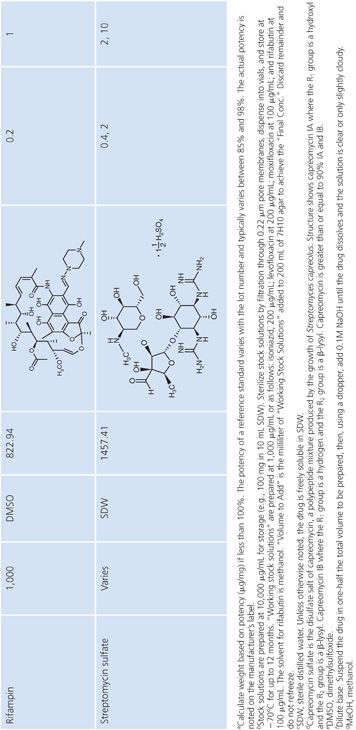
Preparation of Media
Three solid media have been commonly used for M. tuberculosis susceptibility testing: Middlebrook and Cohn 7H10 and 7H11 agar supplemented with oleic acid/albumin/dextrose/catalase (OADC), and Löwenstein-Jensen egg-based medium. All of these media are usually commercially available. The Middlebrook 7H10 agar is preferable because of the simple composition and ease of preparation of this medium. Some resistant isolates of M. tuberculosis may grow more luxuriantly on Middlebrook 7H11 agar, but the concentrations of certain drugs must be adjusted and routine use of Middlebrook 7H11 medium is not encouraged. Egg-based media, including Löwenstein-Jensen, Wallenstein, and Ogawa media, are not recommended for susceptibility testing. However, it is important to note that this recommendation (i.e., to not use Löwenstein-Jensen) is not universally accepted and may not always be practical. Löwenstein-Jensen medium (International Union Tuberculosis Medium [IUTM] modification) with or without INH (0.2 mg/L), rifampicin (40 mg/L), dihydrostreptomycin (4 mg/L), and ethambutol (2 mg/L) incorporated into 28 mL universal containers or screw-capped tubes is recommended by the International Union Against Tuberculosis and Lung Disease for proportion testing of M. tuberculosis (48). Indeed, this medium may be more readily available where the incidence of TB is highest and the International Union procedures have proven to be effective in treating and controlling TB in those parts of the world.
The 7H10 agar medium is prepared according to the manufacturer’s directions. The antimicrobial agents are incorporated into 200-mL aliquots of 7H10 agar held at 50°C to 56°C, following the schedule in Table 5.4. The medium is supplemented with OADC and dispensed (in 5-mL aliquots) into sterile plastic quadrant plates. One quadrant is filled with 7H10 medium without drug, which is for the growth control. The medium should be dispensed quickly, the agar allowed to solidify, and either used immediately or stored at 4°C in sealed plastic bags for not more than 28 days (52). The plates should be protected from light during storage and thoroughly equilibrated to room temperature. The agar surface must be dry before inoculation.
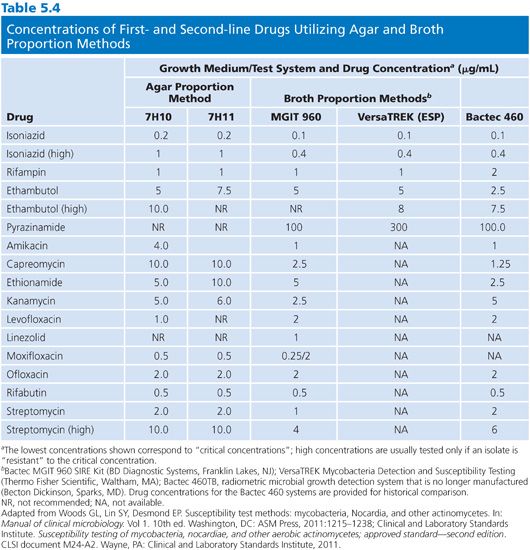
Disk Elution Alternative
The disk elution alternative method for preparing media for the proportion method of susceptibility testing is both convenient and practical. The disk elution method was originally developed by Wayne and Krasnow (53) and was critically evaluated in comparison with an agar dilution method (52,54). Commercially available disks (e.g., BD Diagnostic Systems, BBL Sensi-Disk Antimycobacterial, Cockeysville, MD) impregnated with standardized amounts of first-line antimycobacterial drugs are placed in separate quadrants of sterile plastic plates. The amounts of drug contained in the disks, the distribution of disks into the quadrants of the plate, and the final concentrations of drug are shown in Table 5.5. The disks are aseptically placed in the center of the quadrant and 5 mL of 7H10 agar (without drug) at about 52°C is dispensed into each quadrant. The disks should remain submerged and centered in the quadrant until the medium solidifies. The plates should be incubated overnight at room temperature to allow for complete diffusion of the drug through the medium. Plates containing antimicrobial agents should be used immediately or stored in plastic bags, in the dark, at 4°C for not more than 4 weeks. At 37°C, more than 50% of the initial concentration of INH, ethambutol, rifampin, ethionamide, and cycloserine in agar plates is lost to deterioration in 2 days (ethambutol) to 1 to 2 weeks (52); these values emphasize the need for proper storage of plates.
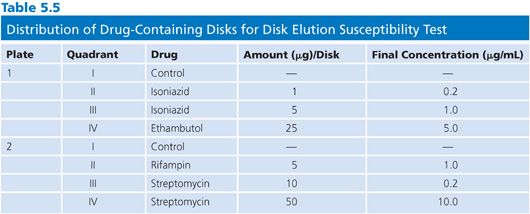
Quality Control Strains
No strains of M. tuberculosis have been as rigorously standardized for controlling the quality of susceptibility tests as are available for testing rapidly growing gram-negative bacilli and gram-positive cocci. However, there are several strains of M. tuberculosis with different resistance phenotypes that can be used for quality control (QC) testing. At least one fully susceptible strain should be considered for QC testing (e.g., M. tuberculosis H37Rv [ATCC strain 27294], which is susceptible to the primary agents). The choice of a resistant strain is more problematic. Many of the resistant strains of M. tuberculosis that are available from ATCC have very high levels of resistance, which is not particularly useful when confirming the ability of a method to distinguish resistant from susceptible. A strain with a stable low-level resistance phenotype to a single drug is preferred for QC strain (e.g., ATCC BAA-812). The BAA-812 strain has a stable mutation in the inhA promoter and consistently yields an MIC for INH between 0.2 and 0.8 μg/mL. Multiple resistant isolates (i.e., MDR M. tuberculosis) should not be used because of the risk of laboratory-acquired infection. The QC strains are grown in liquid medium, diluted to a standard turbidity, dispensed into 1-mL aliquots, and frozen at −70°C. Once each week, or whenever a new lot of medium is prepared, one or more aliquots of the control strains should be thawed and two dilutions prepared according to the standard dilution protocol. One dilution should yield 200 to 300 colonies and the other dilution should yield 20 to 30 colonies on the control plates. In this manner, both the quality of the medium and the dilution technique are tested.
Media Components
Guthertz et al. (55) examined the effects of different lots of Middlebrook 7H10 agar, OADC, and 0.5% glycerol on standard susceptibility test results using a modified proportion method. Three assays were used to measure the comparative quality of the components: (a) a comparative resistance assay to monitor drug stability in solution and in agar, (b) a disk potency assay to monitor the potency of disks impregnated with antimycobacterial agents, and (c) a standard concentration assay to monitor changes in antibiograms caused by changes in the test medium. Rejection criteria included both changes in the size of colonies and changes in the number of colonies; a 20% change in either colony size or number was considered significant. The test strains included M. tuberculosis H37Rv (ATCC strain 27294) and several strains of M. tuberculosis, M. avium, and other slowly growing mycobacteria. By this method, the authors concluded that 30% of lots of OADC and 15% of lots of Middlebrook 7H10 agar were unacceptable, leading to interpretations of both false susceptibility and false resistance. The primary reasons for rejection were reduced colony size and drug binding. This study emphasizes the importance of recording the lot numbers of all components and testing new lots of medium components, especially OADC and 7H10 powder, with standard strains of M. tuberculosis and other slowly growing mycobacteria, to ensure the reliability of results from batch to batch. A convenient protocol for monitoring OADC was described by Butler et al. (56). They established a correlation between the ability of OADC to support the growth of Bacillus subtilis (measured as a change in optical density over 24 hours) in a heart infusion broth supplemented with a test lot of OADC and the ability of OADC to support mycobacterial growth. Acceptable lots of OADC support the growth of B. subtilis (biomass turbidity increase of 0.2 OD650 in 24 hours) and good growth of mycobacteria, whereas failure to support growth of B. subtilis correlates with poor growth of mycobacteria.
Sterility Tests
A representative sample (10%) of each lot of plates (agar dilution or disk elution) should be incubated for 48 hours at 35°C and checked for sterility.
Agar Proportion Method: Direct Test
The principle of the “direct test” is to inoculate drug-containing media directly with a smear-positive, processed (digested, decontaminated, and concentrated) specimen. The advantages of the direct test are decreased time to reporting of susceptibility test results, a potentially more accurate measure of the percentage of resistant tuberculous bacilli in the specimen, and decreased cost. The direct test should be performed only with specimens that are smear-positive for AFB and only using the agar proportion method or a commercial method that has been specifically approved for use as a direct test. The inoculum should be carefully controlled because overinoculation may lead to false resistance and underinoculation may lead to false susceptibility. The direct method may be most appropriate when there is a high prevalence of drug resistance with a patient population, but logically, this would require that second-line drugs be tested as soon as possible or feasible (49).
An agar proportion direct method includes the following steps:
1. Digest, decontaminate, and concentrate the specimen, as appropriate, according to an accepted procedure.
2. Prepare, stain, and examine a smear using either a fluorochrome or carbol-fuchsin method. Record the number of bacilli in each of 20 fields and calculate the average number per field. Because the test is based on measuring a reduction in colony-forming units (CFU), count any clump as a single organism; however, it is important to emphasize that the suspension should be completely homogenized. Dilute the specimen in water (e.g., 0.5 mL of specimen in 4.5 mL of water) based on the stain, using the dilution scheme shown in Table 5.6 as a guide. Choose two concentrations so there is a 100-fold difference between the concentrations of the two inocula.
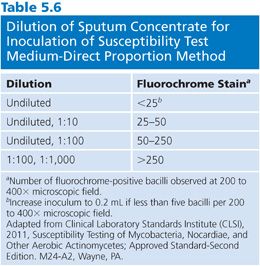
3. Use a sterile safety pipette to inoculate 0.1 mL of each dilution onto each quadrant of duplicate plates and use separate sets of plates for each dilution of the inoculum. Let the plates stand for 1 hour to absorb the inoculum. If the patient has received anti-TB medications, include an undiluted inoculum regardless of the smear results because AFB observed in the smears of specimens from treated patients may be nonviable.
4. Place the plates into CO2-permeable polyethylene bags (6 × 8 inches) with the medium on the bottom, that is, do not invert the plates. Heat-seal the bags and incubate the plates at 35°C to 37°C in 5% to 10% CO2.
5. Read the plates weekly for 3 weeks; however, do not report a result as “susceptible” before 3 weeks. Colonies of “resistant” isolates often develop more slowly than the colonies of susceptible isolates. If growth is not apparent, examine each quadrant with a dissecting microscope (30× to 60× magnification) for the presence of slowly growing microcolonies; however, take care not to overinterpret the results because the deterioration of drugs may lead to the appearance of microcolonies. Grade the results, at both dilutions, according to the following criteria: (a) confluent (too numerous to count), record 3+ or 4+; (b) in the range of 100 to 200 colonies, record 2+; (c) in the range of 50 to 100 colonies, record 1+; (d) less than 50 colonies, record the actual number (note the presence of microcolonies). The control plate, at one dilution or the other, should contain 50 to 100 colonies, and the percentage of resistant colonies is based on this number. If the control plate contains insufficient growth or confluent growth, the test must be repeated unless the isolate is fully susceptible to all drugs tested. The susceptibility test should be terminated at 3 weeks because even susceptible isolates may eventually grow in the presence of bacteriostatic drugs.
6. Retain the control plate (quadrant) as an additional source of the isolate because this plate was directly inoculated with the specimen.
Mycobacteria Growth Indicator Tube
Beginning in 1993, drug susceptibility testing in liquid medium became the standard of practice (57). Because some patients with drug-resistant TB, particularly those with AIDS, were dying before results of solid medium proportion testing became available, an emphasis was placed on the relatively rapid testing methods, including Bactec 12B medium, TREK Diagnostics ESP medium, and others. Bactec 12B medium permitted rapid and accurate drug susceptibility testing of M. tuberculosis complex, but it had several drawbacks including use of radioactive carbon-14 and the requirement to use needles for inoculation, growth detection, and removal of samples for acid-fast smear or other purposes.
For these reasons, Becton Dickinson discontinued the production of Bactec 12B medium and now produces a nonradioactive broth culture system, the Mycobacteria Growth Indicator Tube (MGIT), along with reader/incubator instruments with a capacity for 960 or 320 tubes (cultures). At the bottom of each MGIT broth tube is silicon rubber impregnated with ruthenium pentahydrate, which serves as a fluorescence quenching–based oxygen sensor. When oxygen is depleted due to growth of mycobacteria, this is detected as fluorescence by the automated 960 reader. Fluorescence is measured every 60 minutes by the 960 TB instrument and expressed as fluorescence units unique to this instrument. When primary, pure culture of M. tuberculosis bacilli consumes a sufficient amount of oxygen to generate a defined threshold of fluorescence, the culture is reported as “positive” by the 960 TB instrument. The positive threshold for the 960 TB instrument is equivalent to 105 or 106 CFU/mL of medium.
In 2002, the 960 TB system was approved by the U.S. Food and Drug Administration (FDA) for susceptibility testing of M. tuberculosis complex against the primary drugs: INH, rifampin, ethambutol, streptomycin, and PZA (58). The concentrations of primary drugs were chosen to give results equivalent to previous reference methods, and the 960 TB test performance characteristics were found to be accurate and reproducible (59–61). However, a recent meta-analysis and review of the 960 TB system by Horne et al. (62) found that ethambutol testing may not be equivalent to other methods. In this study, the sensitivity for detecting ethambutol resistance was only moderate, and the investigators suggested that a review and possible revision of the ethambutol test concentration in MGIT 960 TB test may be warranted. This suggestion is supported by data from a review of proficiency testing results, which showed that when isolates were expected to be resistant to ethambutol, based on previous tests, the MGIT 960 TB test reported the isolates as resistant only 79% of the time, a performance markedly poorer than that seen with other drugs (63).
For the MGIT 960 TB test, lyophilized drug preparations are provided, which are reconstituted with water to prepare stock solutions for streptomycin, INH, rifampin, ethambutol (SIRE), and PZA drugs. Although streptomycin is provided in the MGIT SIRE kit, the Clinical and Laboratory Standards Institute (CLSI) M24A2 standard recommends making streptomycin a second-line drug to be tested only on request. MGIT drug susceptibility testing kits from Becton Dickinson include a growth supplement, which is composed of OADC (oleic acid/bovine albumin/dextrose/catalase) in the case of SIRE kits, and OADC supplemented with polyoxyethylene stearate (POES) in the case of PZA test kits. The supplements should not be interchanged between SIRE and PZA test kits.
The source of inocula can be either a pure culture of fresh growth on solid medium or a positive MGIT 960 vial containing actively growing bacilli. Separate protocols are provided for inocula from MGIT broth and solid medium. Careful adherence to the manufacturer’s protocol in preparation of inocula is essential. From MGIT 960 broth, if testing is performed at 1 or 2 days following detection of growth by the 960 instrument, the broth may be mixed and used directly as inoculum for MGIT 960 drug susceptibility testing. However, if testing is delayed 3 to 5 days from the time of initial growth detection, it is likely the concentration of TB bacilli will be too high and the culture must be diluted 1:5 before being used as an inoculum. From solid medium, a suspension is made from fresh growth not more than 14 days after the first appearance of colonies on the medium. The suspension is adjusted to a turbidity equivalent to a McFarland 0.5 standard and then diluted 1:5 using 7H9 broth or MGIT 960 medium.
When primary MGIT broths inoculated with patient specimens are detected by the MGIT 960 instrument as positive, laboratory protocols may vary in terms of the amount of growth removed for acid-fast microscopy, verification of growth purity, and identification (e.g., Gen-Probe AccuProbe, San Diego, CA). Thus, the amount of growth remaining for drug susceptibility testing can vary. For this reason, a proposal was made to use the turbidity-based inoculum technique as described for solid media, with liquid media as well as with solid media (64).
Control vials are inoculated with a 1:100 dilution (for SIRE drugs) or 1:10 dilution (for PZA) of the inoculum in sterile saline. Control and drug-containing test vials are then registered into the reader/incubator system in sets. The growth in each MGIT tube is then monitored by the instrument by periodic measurements of fluorescence. When the control vial fluorescence reaches a level of 400 growth units (GU) within 4 to 13 days, the MGIT system flags the completion of a drug test and interprets the susceptibility or resistance to each drug using a threshold of greater than 100 GU for resistance or less than or equal to 100 GU in the drug-containing tubes to indicate susceptibility.
When growth is detected in a drug-containing MGIT tube, it is important to confirm that it is M. tuberculosis complex and not a non-TB Mycobacterium or other contaminant. Visual inspection of the tube should show clumps settled at the bottom of the tube (Fig. 5.2). Acid-fast microscopy should generally show AFB cording or in tight clumps. When visual or acid-fast observations are not what is expected, a subculture or “purity plate” can be inoculated to confirm whether a contaminant is present. If a culture contains nonmycobacterial contaminants or is suspected to contain non-TB mycobacteria, it may be possible to prepare a pure inoculum of M. tuberculosis complex from the purity plate.
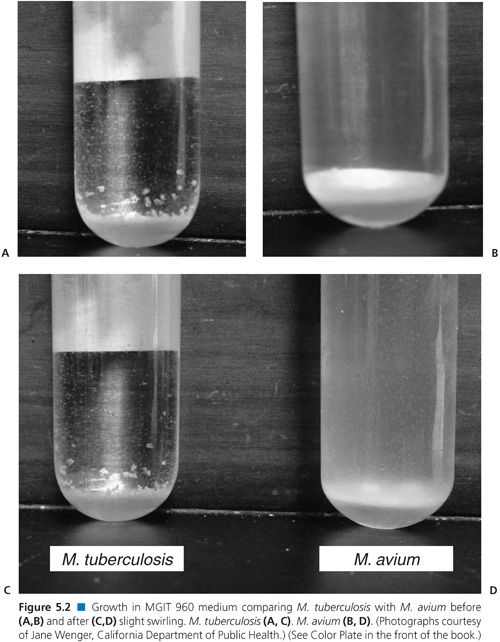
Each laboratory must develop a protocol for how and when to confirm unusual or unexpected drug susceptibility testing results. For example, resistance to rifampin in an isolate which is INH susceptible or monoresistance to ethambutol or PZA may be questionable and indicate the need for retesting, preferably by a different method. Alternative methods could include testing in agar medium by the proportion method or molecular methods such as DNA sequencing or use of DNA probes to detect common resistance-conferring mutations. If an alternative method is not available, repeat testing by the same method may sometimes be expected to determine whether an error has been made. Although confirmatory testing is underway, the health care provider caring for the patient should be made aware of preliminary results and the timing of expected confirmatory testing.
Protocols have been developed for testing TB complex isolates for susceptibility to second-line drugs as well (64–66). These and other studies were considered in the preparation of a consensus document by the WHO, which makes recommendations for test concentrations for first- and second-line drugs in various media including Middlebrook agars, Löwenstein-Jensen medium, and MGIT (67). For MGIT, critical test concentrations (μg/mL) recommended are 1.0 for amikacin, 2.5 for capreomycin, 2.0 for levofloxacin, 0.25 for moxifloxacin, 5.0 for ethionamide, and 1.0 for linezolid. Testing levofloxacin or moxifloxacin is recommended in order to encourage the use of these fluoroquinolones, which are more active than ofloxacin against M. tuberculosis. Testing of moxifloxacin at the WHO-recommended concentration of 0.25 μg/mL has been shown to correlate with the presence of mutations in the quinolone resistance-determining region (QRDR) of the gyrA gene and to predict resistance to ofloxacin (32). However, moxifloxacin may retain clinical activity despite the presence of some mutations associated with MICs less than 2 μg/mL. Clinical evidence for use of moxifloxacin for treatment of cases in which the isolate is resistant at a concentration of 0.25 μg/mL but susceptible at 2 μg/mL is slight (68), but treatment using high-dosage moxifloxacin may be considered for the most difficult to treat cases.
PZA testing is a particular challenge by culture-based methods (69,70). PZA is active in acidic environments, so its activity is evaluated in an acidified version of MGIT broth at a pH at or near 6 where growth of M. tuberculosis is suboptimal. Precise adherence to inoculum protocol is critical for PZA since the inoculum can influence pH of the medium. Piersimoni et al. (71) have recommended a reduced inoculum volume of 0.25 mL rather than 0.5 mL and presented data indicating that a reduced inoculum leads to more accurate results (71). Another factor is the age of the culture because PZA has been shown to be more active against older cultures than it is in inhibiting log phase cultures (70). A further confounding factor may be inhibition of some strains by the POES incorporated into the MGIT test system (72). These variables render culture-based testing of PZA in MGIT or other media less reliable than the testing of INH or rifampin. A test of susceptibility to nicotinamide at a neutral pH has been proposed as an alternative, but sequencing of the pncA gene is more promising because of quick and reliable results (69,72). Assay for pyrazinamidase activity has also been shown to predict PZA resistance with a sensitivity of approximately 89% and a specificity of 97% or greater (73,74).
Other Culture-Based Commercially Available Systems for Drug Susceptibility Testing of M. tuberculosis in Liquid Medium
ESP/VersaTREK
TheVersaTREK system, formerly known as ESP, is available for testing susceptibility of isolates of M. tuberculosis complex for susceptibility to INH, rifampin, ethambutol, streptomycin, and PZA. It gives results similar to MGIT 960 (75–77). The VersaTREK system is an automated broth culture system with detection of growth by means of detecting changes in gas pressure in the head space over the culture broth. The PZA concentration (300 μg/mL) is higher than that used in MGIT, and in the study by Espasa et al. (77), VersaTREK gave slightly fewer resistant results than MGIT.
Sensititre MYCOTB Minimal Inhibitory Concentration Panel
This system is also manufactured by TREK Diagnostics. It tests susceptibility of M. tuberculosis complex isolates using a 96-well microtiter plate format to three first-line (INH, rifampin, ethambutol) and nine second-line (amikacin, cycloserine, ethionamide, kanamycin, moxifloxacin, ofloxacin, PAS, rifabutin, and streptomycin) drugs. This selection of drugs offers the advantage that two drugs are tested in the fluoroquinolone class, two aminoglycosides, and two rifamycin drugs. It is therefore not necessary to rely on a “class drug” to predict susceptibility or resistance to another drug in the same class. This has several possible advantages. For example, moxifloxacin may retain activity in spite of the presence of a gyrA mutation which causes resistance to ofloxacin, amikacin may still have activity and be useful in the treatment of infections by strains which are resistant to kanamycin due to G-10A mutations in the eis promoter region (78), and rifabutin may be active against some strains of M. tuberculosis which have rpoB mutations which make them resistant to rifampin.
There is controversy regarding the reporting of MIC results for susceptibility testing of M. tuberculosis complex. Some clinicians may be unprepared to interpret MIC results and apply them to decision making regarding design of treatment regimens for TB. There is a danger that clinicians may be unaware of the significance of intracellular infections by TB bacilli or of the presence of special populations of TB bacilli in walled-off lesions and in varying metabolic states. Comparisons of MIC values with serum or tissue achievable levels, as is done for many types of extracellular infections, may be poorly predictive for treatment of TB (79). On the other hand, MIC values may assist a well-informed clinician in making decisions about whether to use rifabutin versus rifampin in some patients whose TB bacilli have an rpoB mutation or whether to use moxifloxacin, perhaps at a higher dose, in the presence of some gyrA gene mutations.
Two studies evaluating the Sensititre system have recently been published, which were supported by the manufacturer (80,81). These studies compared Sensititre results with those obtained using the agar proportion method. When Sensititre drug concentrations which match closely the drug concentrations in agar are chosen for interpretation, high levels of correlation are seen with the agar proportion method, ranging from 94% to 100% for different drugs. More studies are needed to establish the accuracy, reproducibility, and utility of this method. Reading of end points is performed using a mirror or video camera linked to a computer screen.
Microscopic Observation Drug Susceptibility Method
Hardy Diagnostics manufactures a microscopic observation drug susceptibility (MODS) test kit, which uses a 24-well plate with Middlebrook 7H9 broth and OADC supplement. Detection of M. tuberculosis complex is performed starting with decontaminated, concentrated sputum sediments. To make the broth medium more selective for M. tuberculosis complex, a selective “NAPTA” (nalidixic acid, azlocillin, polymyxin B, trimethoprim, amphotericin B) antibiotic solution is mixed with the specimens at the time of inoculation. Drug-free wells serve as growth controls, and specimens are also inoculated into wells containing final concentrations of 0.4 μg/mL INH and 1 μg/mL rifampin. The culture plates are sealed with a “safety lid” and incubated at 37°C. A CO2 atmosphere is not required.
As indicated by the name of this procedure, growth is detected by microscopic observation using an inverted microscope. Growth of M. tuberculosis is observed as cords after approximately 5 to 10 days incubation. Mycobacterium chelonae may also exhibit cording in broth growth but will typically grow in less than 5 days.
MODS can also be performed using reagents, drugs, media, and supplies from various sources in order to reduce cost. MODS requires substantially less time (5 to 10 days) than culture in solid medium followed by drug susceptibility testing in solid medium, which often requires approximately 2 months to complete. MODS, therefore, permits much earlier changes in patient therapy when drug resistance is detected (82–84). Detailed assistance with the protocol may be obtained online at http://www.jove.com/video/845/the-mods-method-for-diagnosis-tuberculosis-multidrug-resistant.
A recent study has tentatively established test concentrations for second-line drugs in the MODS assay (85). The drugs included capreomycin, ciprofloxacin, cycloserine, ethambutol, ethionamide, kanamycin, PAS, and streptomycin. Further testing is required before the test concentrations proposed by this study can be confirmed.
Colorimetric Redox Indicator Methods
Colorimetric redox (reduction/oxidation) methods have been developed to assist the detection of M. tuberculosis growth in drug susceptibility testing, allow resistance to be reported earlier, and standardize reporting. Redox indicators have been proposed for use in low-resource countries, where more expensive systems such as MGIT may not be considered affordable. Broth or agar media are used, with standardized inoculum and drug concentrations (86,87), and a redox indicator such as alamar blue, MTT [3(4,5-dimethylthiazole-2-yl)-2,5-diphenyltetrazolium-bromide], or resazurin. Commonly, a microtiter plate broth culture format is used, with drug-free control wells and drug-containing wells. After incubation for a few days, the redox indicator is added, and growth is detected as a color change. The additional step of adding the redox indicator and the potential biohazard involved with opening a culture following incubation may make these methods less attractive for laboratories which can afford MGIT or other system which permits detection of growth in a sealed tube or plate. However, the fact that media and reagents are nonproprietary may lead to lower prices for redox methods. Martin et al. (86) evaluated a microtiter plate assay with resazurin indicator for detection of extensively drug-resistant TB in a multicenter study. Critical concentrations were 0.5 μg/mL for rifampin, 0.25 μg/mL for INH, 2 μg/mL for ofloxacin, and 2.5 μg/mL for kanamycin and capreomycin. Overall accuracy figures for the five drugs, compared with the Löwenstein Jensen proportion method, were 98.4%, 96.6%, 96.7%, 98.3%, and 90%, respectively.
NONTUBERCULOUS MYCOBACTERIA
The nontuberculous mycobacteria have been divided historically into two groups: (a) slowly growing mycobacteria with generation times of approximately 24 hours and that take more than 7 days to form visible colonies on solid media and (b) rapidly growing mycobacteria (RGM) with generation times less than 24 hours and that form visible colonies in 7 days or less. However, these historical distinctions are not absolute. Slowly growing mycobacteria can fluctuate in growth rate from a latent nonreplicating state to a comparatively fast rate of replication in patients with active disease. Even RGM may take much longer than 7 days for growth to be detected during primary isolation (the definition of rapidly growing refers more specifically to the growth on solid medium inoculated from a dilute suspension from a primary culture). Nevertheless, the terms rapidly and slowly growing mycobacteria continue to be used and continue to have relevance to the diagnosis and treatment of mycobacterial infections. Indeed, taxonomic studies based on sequence analysis (e.g., 16S rRNA, hsp65, rpoB, and sod genes) have validated the phenotypic classification of slowly and rapidly growing mycobacteria (11).
Based on molecular taxonomic methods, there are more than 120 species of nontuberculous mycobacteria, but the majority (90%) of infections in humans are caused by two species of slowly growing mycobacteria (MAC and Mycobacterium kansasii) and three species of RGM (Mycobacterium fortuitum, M. chelonae, and Mycobacterium abscessus) (8,88,89). The two slowly growing mycobacteria are important causes of pulmonary disease; MAC is the predominant cause. The RGM species are important causes of skin and soft tissue, pulmonary, and nosocomial infections, especially following catheter insertions, augmentation mammaplasty, and cardiac bypass surgery (90). Disseminated MAC disease was an important coinfection in HIV-infected patients prior to the advent of effective antiretroviral therapy. Disseminated disease caused by RGM is rare and is usually associated with immunodeficiency, including that associated with corticosteroid therapy, but not HIV infection (91).
The ATS in conjunction with the IDSA established diagnostic criteria for nontuberculous mycobacterial lung disease: (a) chest radiograph or high-resolution computed tomography scan, (b) three or more sputa positive for acid-fast bacteria, and (c) exclusion of TB or other disorders. However, even these criteria are most applicable to disease caused by MAC, M. kansasii, and M. abscessus and application of the criteria to other nontuberculous mycobacteria may not be appropriate (10).
There are a variety of antimicrobial agents recommended for the treatment of infections caused by nontuberculous mycobacteria (Table 5.7) (88,89,92). However, the activity of these antimicrobial agents varies considerably from species to species, which reflects differences in inherent and acquired resistance. The different mechanisms of resistance include (a) lack of cell wall penetration; (b) biotransformation by ribosylation, acetylation, nitrosation, and hydrolysis; (c) induction of inactivating enzymes; (d) presence of efflux pumps; and (e) mutation of the gene that encodes the structural or enzymatic target. Therefore, drug susceptibility testing is often an important component of a successful treatment strategy. The mechanisms of resistance to antimicrobial agents in nontuberculous mycobacteria are both the same and different compared with the M. tuberculosis complex. Most of these mechanisms of resistance are discussed in greater detail elsewhere in this chapter, but a summary of the mechanisms of resistance that are specific to nontuberculous mycobacteria are shown in Table 5.8. The role of the mycobacterial cell wall and the presence of porins, efflux pumps, and cell wall maintenance mechanisms appear to be important sources of “inherent” antimicrobial resistance among the nontuberculous mycobacteria, perhaps to a greater extent than in the M. tuberculosis complex (93).
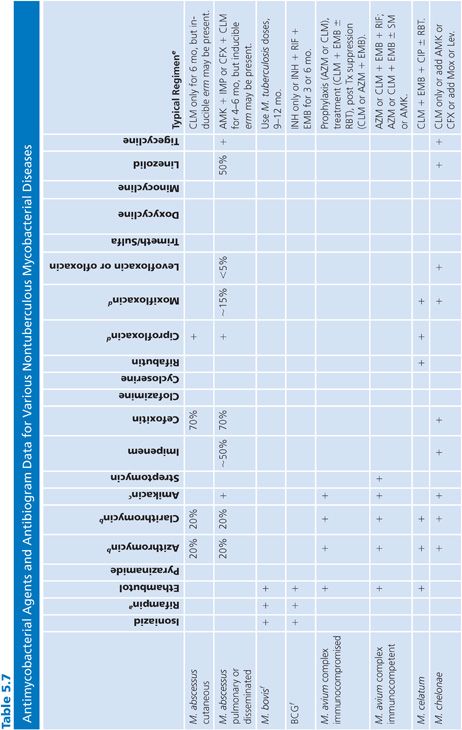
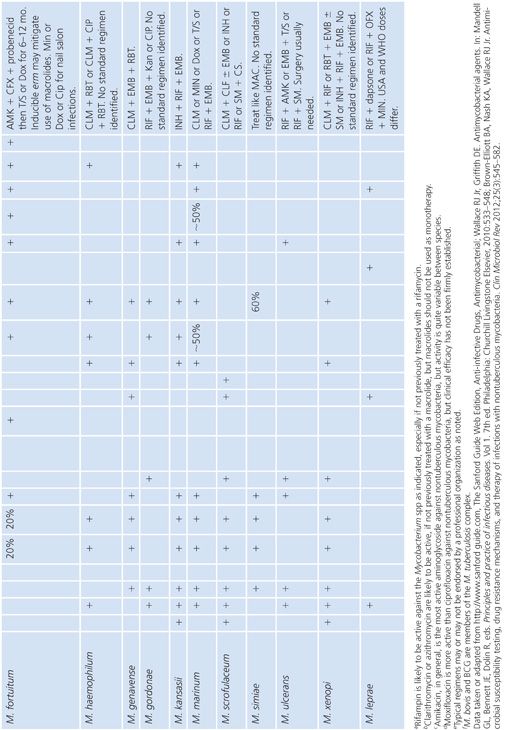
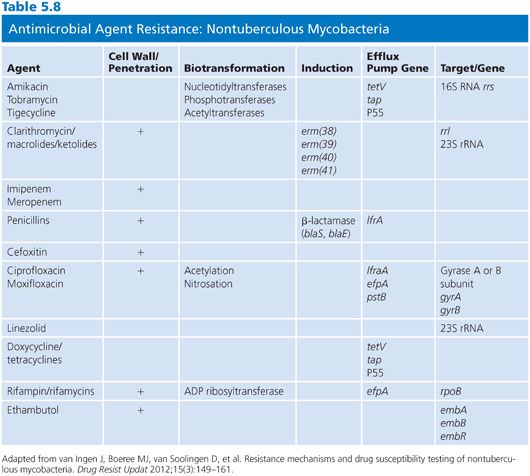
The newer macrolides (clarithromycin, azithromycin, roxithromycin) and perhaps the ketolides are important drugs for the treatment of many nontuberculous mycobacterial infections, for example, M. chelonae and M. abscessus and perhaps 80% of M. fortuitum (89). However, the apparent susceptibility of the RGM to macrolides may be misleading as several harbor an inducible erm gene (e.g., erm [39]), that can confer a high-level clarithromycin resistance (94,95). In addition, most isolates of M. chelonae have clarithromycin MICs of 0.25 μg/mL or less, but resistance develops quickly with monotherapy (96); in these cases, resistance is conferred by mutation in the 23S rRNA gene (97).
RAPIDLY GROWING MYCOBACTERIA SUSCEPTIBILITY TESTING
It is important to quickly distinguish the RGM from other mycobacteria because all first- and most second-line antituberculous drugs are ineffective against the RGM (88,89,92), which may not always be appreciated by the clinician with limited experience in treating these infections. As previously noted, a more difficult consideration is assessing the clinical significance of RGM isolated from clinical specimens. The nontuberculous mycobacteria that commonly cause most clinical disease are M. abscessus, M. fortuitum group, M. kansasii, M. chelonae, Mycobacterium haemophilum, Mycobacterium ulcerans, Mycobacterium terrae complex, and Mycobacterium marinum (8–10,98). The following species are rarely a cause of clinical disease: Mycobacterium gordonae, Mycobacterium mucogenicum, Mycobacterium botniense, Mycobacterium cookie, Mycobacterium chlorophenolicum, Mycobacterium frederiksbergense, Mycobacterium hodleri, and Mycobacterium murale (98).
In order to optimize the susceptibility testing and facilitate interpretation of the RGM susceptibility results, the CLSI recommends (99) that isolates be identified to at least differentiate the M. fortuitum group from the M. abscessus–chelonae group. Preferably, identification should be to the species level. Several methods have been described for measuring the in vitro susceptibility of RGM, including (a) agar dilution, (b) agar disk elution, (c) Etest, (d) disk diffusion, and (e) broth microdilution (98,99). However, the standard recommended by the CLSI is a broth microdilution assay using cation-supplemented Mueller-Hinton broth (CSMHB) plus 5% OADC supplement as the preferred medium (98,99) and only this method is discussed further. Dry, microdilution plates are commercially available, on a research use only basis, for testing rapidly growing and slowly growing nontuberculous mycobacteria, that is, RAPMYCO and SLOMYCO, TREK Diagnostic Systems (Fig. 5.3).
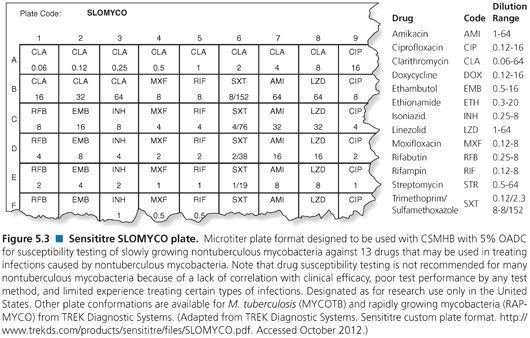
Antimicrobial Agents
Antimicrobial standard reference powders can be obtained commercially from the manufacturer, from United States Pharmacopeia or the Zentrallaboratorium Deutscher Apotheke. Drug stock solutions, based on the potency of the drug, should be prepared as specified by the CLSI procedure (99) or as specified by the manufacturer.
The agents that should be tested are shown in Table 5.9. Clarithromycin is considered a class representative for newer macrolides (e.g., azithromycin and roxithromycin). Test both ciprofloxacin and moxifloxacin because the spectrums of activity are not identical. Testing of carbapenems can be problematic and should be performed to experienced laboratory, but even then the results are often difficult to reproduce. Imipenem, meropenem, and ertapenem should be tested separately; imipenem is the most active carbapenem against RGM.
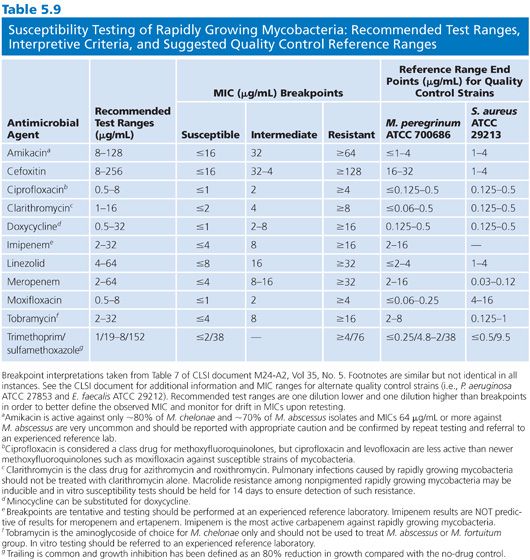
The drug-containing 96-well plates can be prepared fresh for each susceptibility assay or batch prepared ahead of time and stored until needed. Two approaches are recommended for the batch production and storage of drug-containing plates.
1. Drug dilution series can be prepared in broth and 0.1-mL aliquots added to the wells of a 96-well microplate; the plates should then be sealed in plastic bags and stored at −70°C for up to 6 months. The plates should not be stored in a frost-free freezer (such freezers have defrost cycles), and thawed plates should not be refrozen.
2. Drug dilution series can be prepared in sterile distilled water (or other suitable diluent—should not significantly affect the constituents of the broth medium when reconstituted), aliquoted, and the material lyophilized in situ. With this approach, the amount a drug added per well should give the required concentration in a final volume of 0.1 mL.
The commercially available RAPMYCO, dry, extended shelf life microtiter plates include the following drugs: amikacin, amoxicillin/clavulanic acid, cefepime, cefoxitin, ceftriaxone, ciprofloxacin, clarithromycin, doxycycline, imipenem, linezolid, minocycline, moxifloxacin, tigecycline, tobramycin, and trimethoprim-sulfamethoxazole.
The CLSI guidelines are based on the use of flat-bottomed 96-well microplates, which allows the quantitation of microcolonies or bacterial clumps in wells with low-level growth. This ability may aid in determining the end point for some antimicrobial agents. Alternatively, “U”- or “V”-bottomed plates can be used. The benefit of these styles of plate is that all the sedimented bacteria collect in a small area, which helps distinguish wells with low-level growth from wells with no growth. The disadvantage of “U”- or “V”-bottomed wells is that quantitation of microcolonies or bacterial clumps is not feasible.
Inoculation
Susceptibility tests should be performed only on pure cultures. The organisms can be stored on Löwenstein-Jensen slants before testing and then subcultured to a nonselective medium such as trypticase soy or blood agar plates and incubated in air for 2 to 4 days at 30°C to 35°C to obtain discrete colonies. A sterile swab should be used to transfer a sweep of organisms to tubes containing 4.5 mL of sterile water to give a turbidity equivalent to a McFarland no. 0.5 standard. To aid the dispersion of bacteria clumps, 3-mm glass beads (7 to 10 should be sufficient) should be added to the tube and the suspensions vortexed aggressively for 15 to 20 seconds. Any remaining large bacterial clumps should be allowed to settle. RGM that are adjusted to a McFarland no. 0.5 standard contain 1 × 107 to 2 × 108 CFU/mL (100).
The final inoculum preparation depends on the nature of the drug-containing plates. (a) If plates containing lyophilized agents are used, then the bacterial suspension described earlier must be diluted 200-fold to give a final density of approximately 5 × 105 CFU/mL, for example, 50 μL of suspension (turbidity equivalent to a McFarland no. 0.5 standard) mixed with 10 mL of CAMHB broth for RGM or 10 mL CAMHB plus 5% OADC for slowly growing nontuberculous mycobacteria. Aliquots of 0.1 mL per well (approximately 5 × 104 CFU) are then dispensed into each well of the assay plates. (b) If the plates contain drugs already reconstituted in growth medium (0.1 mL per well), the inoculum should be diluted to a density of approximately 5 × 105 CFU/mL. Aliquots of 0.1 mL per well (approximately 5 × 104 CFU) are then dispensed into the assay plate. Appropriate adjustments should be made if multipronged inoculators are used to deliver an inoculum of 0.01 mL per well. The plates are sealed in plastic bags or placed in another sealed storage container and incubated at a temperature appropriate for the test microorganism. Inoculate a nutrient agar plate to check for purity of the inoculum.
Reading of Results
The plates should be examined after 3 days (72 hours) and then daily up to day 5 (120 hours). Usually, the MICs can be read at day 3 with most M. fortuitum group isolates. In contrast, some strains of M. chelonae may require an incubation time of 4 days (96 hours). Minimally, the no-drug controls should be visibly turbid with clumps of bacteria at the bottom of the wells. The most reliable results are likely to be obtained with the shortest incubation period that gives acceptable growth in control wells. This is especially important for drugs that are unstable in broth media, for example, imipenem. It is advisable to repeat the testing of isolates that have MIC interpreted as being indicative of resistance, either in-house or sent out to a reference laboratory. Testing carbapenems, tetracyclines, and tigecycline may be problematic because of drug instability. Isolates that test susceptible to clarithromycin should be incubated for a total of 14 days to assure detection of inducible macrolide resistance (94,95).
The MIC is the lowest concentration of antimicrobial agent that completely inhibits the growth of the organism as detected by the unaided eye. End points are easy to read for most drugs; however, a faint haze of growth is common with sulfonamides. Consequently, the sulfamethoxazole MIC is usually determined from the well showing approximately 80% inhibition of growth compared to the no-drug control well.
Quality Control
QC procedures should reflect regulatory requirements and acceptable standards of practice. Well-characterized reference strains should be used for QC, for example, Mycobacterium peregrinum ATCC 700686, Staphylococcus aureus ATCC 29213, Pseudomonas aeruginosa ATCC 27853, and Enterococcus faecalis ATCC 29212. The importance of inoculum preparation cannot be overemphasized because overinoculation may result in false resistance and underinoculation in false susceptibility. QC strains should be set up at least weekly for laboratories that perform more than one test per week. In laboratories performing fewer tests, the QC strain should be always included. With stored drug-containing microplates, each batch should be validated with the QC strain and tested for sterility before use.
As with all clinical tests, reliability and reproducibility are critical. Thus, laboratory personnel who score susceptibility assays should be monitored by comparing end points with that determined by an experienced reader. All personnel should agree ±1 dilution with the experienced reader.
MYCOBACTERIUM AVIUM COMPLEX
The MAC is traditionally defined as a serologic complex divided into 30 or more serovars (101) based on the composition of the cell surface oligosaccharide linked to a peptidoglycolipid core that is produced by all members of the complex (102). In the postgenomic era, the taxonomy and clinical significance of MAC is more complex but better understood. It is now clear that the MAC includes both strictly environmental mycobacteria and host-associated pathogenic mycobacteria with specific genetic distinctions. In taxonomic terms, M. avium and Mycobacterium intracellulare are distinct species and the MAC consists of a single species, M. avium, with multiple subspecies, notable M. avium subsp avium, M. avium subsp paratuberculosis, and M. avium subsp silvaticum (103,104). M. avium subsp. avium is a cause of avian TB, cervical lymphadenitis in children (scrofula), chronic pulmonary disease in cystic fibrosis patients and elderly women, and the cause of disseminated disease in AIDS patients with extremely depleted T cells (105,106). M. avium subsp paratuberculosis is an important animal pathogen and the cause of Johne’s disease in ruminants and M. avium subsp silvaticum cause disease in wood pigeons. M. intracellulare causes pulmonary disease in immunocompetent humans and has been isolated from animals and from the environment. Drug susceptibility testing has focused on M. avium subsp avium and to a much lesser degree on M. intracellulare.
Mycobacterium avium Complex Resistance
MAC isolates are predictably resistant to INH and only variably susceptible to rifampin and ethambutol, and the susceptibility patterns are considerably more variable than those of M. tuberculosis (107,108), emphasizing the potential importance of susceptibility testing. The inherent antimicrobial resistance is most likely due to the impermeability of the MAC cell wall and membrane (109), and in vitro cell-free studies confirmed that drug targets (e.g., ribosomes, ribosomal subunits, and RNA polymerase) in MAC cells bind the drugs and the corresponding target functions are inhibited. MAC isolates, like most mycobacteria, produce low levels of β-lactamase (110), but there is no evidence that MAC isolates actively degrade or inactivate β-lactams or possess inactivating enzymes for other antimicrobials. Most MAC isolates have plasmids of varying size and, while plasmids have been associated with antimicrobial resistance in some MAC isolates, specific resistance transfer factors have not been identified (111,112).
Colony Variants
Susceptibility testing of the MAC is complicated by the observation that MAC isolates display two colony variants on agar-based media (113). One colony variant is flat, spreading, and translucent in appearance, while the second colony variant is raised, condensed, and opaque (Fig. 5.4). The translucent variant is more resistant to antimicrobial agents (113) and is more virulent in animal models of infection (114). Stormer and Falkinham (115) showed that nonpigmented variants of M. avium, isolated from both the environment and patients with disseminated M. avium disease, are significantly more resistant to a variety of antimicrobial agents than are pigmented segregants of the same strains. Because the pigmented and more susceptible variants appear more quickly and are more prominent on culture plates, nonpigmented variants could be overlooked in the selection of M. avium colonies for susceptibility testing.
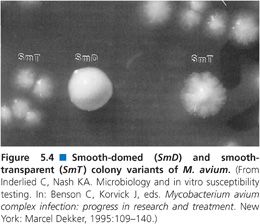
When to Test
In most situations, it is unnecessary to perform in vitro susceptibility tests on initial MAC isolates. However, establishing baseline MIC values may assist in interpreting susceptibility test results weeks or months after the start of therapy. Testing is appropriate for patients on prior macrolide therapy or patients who develop bacteremia while on macrolide prophylaxis for MAC. Also, susceptibility testing may be useful if a patient relapses or if the infection is intractable and the clinical situation desperate. Testing for macrolide resistance may help in deciding whether to add drugs to a treatment regimen. However, it is not clear whether the macrolide should be withdrawn in the face of resistance. Little is known about the effects of multiple drug regimens on such resistant strains, and the chance of polyclonal MAC infections leads to the possibility of mixed susceptible and resistant strains (3). Susceptibility testing should be repeated 3 months after the start of treatment for disseminated disease or after 6 months for patients with pulmonary disease (116).
Antimicrobial Agents
While MAC infections are usually treated with a combination of antimicrobial agents, in vitro susceptibility testing is often restricted to clarithromycin and perhaps moxifloxacin and linezolid. There is animal (117,118) and limited human data (119) on the use of mefloquine to treat MAC infections in humans (120), but no clinical trials have been performed to validate in vitro susceptibility testing methods or interpretive criteria for these agents. CLSI recommends that M. avium isolates be tested if isolated from patients previously treated with a macrolide; if isolated from blood during prophylaxis; from patients with culture-positive relapse; and clinically significant isolates at baseline or to hold such isolates for future testing.
Methods
MAC is most commonly tested using a broth microdilution method and Mueller-Hinton broth supplemented with cations and 5% OADC and adjusted to pH 7.3 or 7.4 as recommended by the CLSI (99). The 2011 CLSI document also recommends use of Bactec 12B medium and the Bactec 460 instrument, but the Bactec 12B medium is no longer available (98). Clarithromycin is the only drug that should be routinely tested. MAC also has been tested using SLOMYCO Sensititre Panels and JustOne Strips (TREK Diagnostic Systems, Cleveland, OH), but these latter methods are designated as “for research use only” and neither method has been evaluated in multisite studies (Fig. 5.3) (121). The SLOMYCO microtiter plates are designed to test a variety of slowly growing mycobacteria and includes several drugs, including clarithromycin (0.06 to 64 μg/mL). As the name implies, the JustOne Strips only test clarithromycin (0.12 to 128 μg/mL).
Inoculum
Collect colonies (especially transparent colony variants, if present) from the surface of a Middlebrook 7H11 plate and suspend in 5 mL of sterile deionized water to match a McFarland no. 0.5 turbidity standard. Transfer 25 μL of the suspension to 5 mL of Middlebrook 7H9 broth with casein or Mueller-Hinton broth with 5% OADC to yield ~5 × 105 CFU/mL in each well of a microtiter plate.
Broth Microdilution Method
Microtiter trays should be inoculated (100 μL/well) within 30 minutes of preparing the inoculum. The trays are sealed with adhesive and incubated at 35°C in ambient air. The plates are first read at 7 days but read with interpretation only when there is sufficient growth in the growth control well. If necessary, the plates are incubated for an additional 7 days. The MIC is defined as the lowest concentration of drug necessary to inhibit visible growth. Interpretive criteria for clarithromycin are shown in Table 5.10 along with tentative interpretive criteria for moxifloxacin and linezolid.
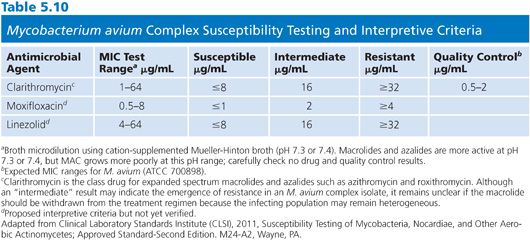
Quality Control
M. avium (ATCC 700898) is clarithromycin-susceptible and recommended for QC. QC strains should be stored at −70°C or for 3 months at −20°C or at ambient temperature for 30 days and subcultured each week or at the time of testing. M. avium ATCC 700898 can be used for quality control and should yield a clarithromycin MIC of 1 to 4 μg/mL; M. avium ATCC 8-700897 is clarithromycin-resistant.
MYCOBACTERIUM KANSASII
M. kansasii is closely related to Mycobacterium gastri; however, the isolation of the former is nearly always clinically significant, while isolation of the latter is rarely of clinical importance (103). In general, the incidence of M. kansasii disease is low and usually responds well to therapy (122,123). M. kansasii isolates from patients who have not been previously treated with rifampin are likely to be susceptible to rifampin at 1 μg/mL (124), and infections have been successfully treated with a combination of rifampin, INH, and a third agent (e.g., ethambutol) (125,126). Rifabutin should be substituted for rifampin in HIV-infected patients treated with a protease inhibitor (127). Clarithromycin can be used in place of INH. M. kansasii isolates can be tested using the proportion method or a broth microdilution method, but the latter is recommended by the CLSI. Initial drug testing should be limited to rifampin and clarithromycin, since treatment failure is mostly associated with rifampin resistance and most M. kansasii isolates will test resistant to INH at 0.2 μg/mL and many test resistant to 1.0 μg/mL (116). INH MICs for M. kansasii are 0.5 to 5 μg/mL, which makes testing somewhat problematic but helps explain the good efficacy of treatment regimen that include INH. Treatment failure is associated with rifampin and/or clarithromycin resistance; thus, testing of initial isolates is recommended. Alternative drugs are listed in Table 5.11.
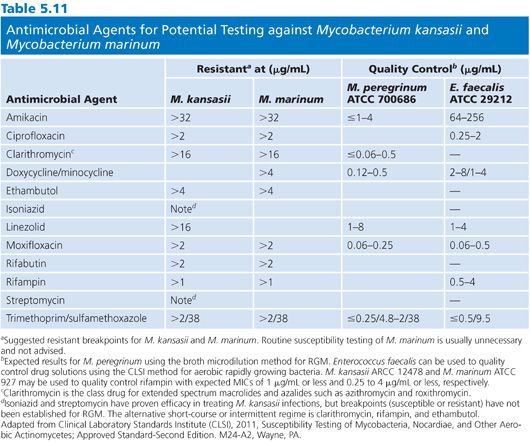
MYCOBACTERIUM XENOPI
Pulmonary infections caused by Mycobacterium xenopi have been described, and the disease occurs more frequently in immunocompromised patients (103,123). Extrapulmonary infections are rare. Pseudo-outbreaks of M. xenopi infections have been reported and the significance of the isolation of this species, especially from a nonsterile body site, should be carefully examined (128,129) because M. xenopi can be mistakenly identified as M. avium if only biochemical tests are used for identification. In vitro susceptibility test results appear to be important in the management of this disease (130); however, the correlation between in vitro susceptibility test results and therapeutic response has been reported to be inconsistent (124). Some indicate that M. xenopi is susceptible to INH, rifampin, streptomycin, and cycloserine, while others dispute these results (131). A treatment regimen of clarithromycin, rifampin, and ethambutol is recommended by the ATS/IDSA (10). The CLSI recommends that in vitro susceptibility test of M. xenopi follow the guidelines for M. kansasii (see earlier discussion) (99), although M. xenopi is reported to not grow well in CSMHB with OADC. M. xenopi also is considered a thermophile and grows better at 42°C to 45°C.
MYCOBACTERIUM SZULGAI
Mycobacterium szulgai is a scotochromogen at 37°C and a photochromogen at 25°C and was first reported to cause pulmonary disease in the early 1970s. Along with the other uncommon species of slowly growing, nontuberculous mycobacteria, M. szulgai appears to cause disease primarily in persons with a history of chronic lung disease (99,123). M. szulgai is not considered an environmental Mycobacterium and isolation from clinical specimen is probably always significant (99). These infections are reported to respond to INH, rifampin, and ethambutol (131); Woods and Washington (124) characterized M. szulgai as only slightly more resistant than M. tuberculosis to anti-TB agents, and they suggested that streptomycin, capreomycin, and viomycin are potential alternatives to the three previously mentioned first-line agents.
MYCOBACTERIUM MALMOENSE
Mycobacterium malmoense is a nonpigmented environmental Mycobacterium that is closely related to Mycobacterium shimoidei, but in clinical laboratories, it may be more important to distinguish M. malmoense from M. gastri and M. terrae because of the difference in clinical significance (103). M. malmoense has been reported as a frequent cause of pulmonary infection mostly in elderly patients with underlying lung disease, including TB and malignancy, but disseminated disease was reported in an HIV-infected patient (123,132). M. malmoense is second to M. avium as the most common cause of cervical lymph node infections in children (10). Although M. malmoense is reported to be variably susceptible to antimycobacterial agents, it is generally considered to be more susceptible than M. avium. Although there are some recommendations for treatment (133) and there are conflicting reports regarding the susceptibility of M. malmoense to INH (134) and rifampin (131), Hoffner et al. (135) showed that combinations of ethambutol with aminoglycosides, quinolones, or rifamycins were synergistic against M. malmoense. This observation is in agreement with reports on the clinical effectiveness of ethambutol, rifampin, and INH in combination in the treatment of pulmonary disease (136). Banks and Jenkins (133) showed that, although M. malmoense was resistant to rifampin and ethambutol, all strains were susceptible to the combination of these drugs at the lowest concentration.
MYCOBACTERIUM SIMIAE
Mycobacterium simiae is an environmental mycobacterium and an uncommon cause of disease. It is regarded as highly resistant to antimycobacterial agents, perhaps with the exception of ethionamide and cycloserine (124); however, there are exceedingly few cases of disease caused by M. simiae on which to base any firm conclusions about susceptibility to antimycobacterial agents. In an animal test system, clarithromycin in combination with ethambutol and perhaps a quinolone such as ofloxacin was potentially effective (137). As with M. szulgai, disease in humans appears to occur mostly in persons with a history of chronic lung disease, and persons with pulmonary lesions are probably predisposed to colonization with potentially pathogenic environmental mycobacteria (103).
MYCOBACTERIUM MARINUM
This photochromogen is a cause of skin, joint, and deeper infections, primarily of the hand or limbs; infection is usually associated with exposure to water (138,139). The optimum growth temperature for M. marinum is 30°C to 35 °C. The successful management of M. marinum infections requires rapid diagnosis and the avoidance of steroid treatments (138,139). M. marinum is largely considered predictably susceptible to rifampin, rifabutin, and ethambutol, although most infections spontaneously resolve or respond to localized treatment without chemotherapy. Disseminated cutaneous infections respond to rifampin and ethambutol; alternative agents are tetracycline, doxycycline, minocycline, trimethoprim-sulfamethoxazole, ciprofloxacin, and clarithromycin (140,141). M. marinum is resistant to INH and PZA. Routine susceptibility testing using methods and interpretive criteria described for M. tuberculosis is inappropriate, and the methods and interpretive criteria for testing RGM are more likely to provide clinically useful results. Drug susceptibility testing should be restricted to treatment failures.
MYCOBACTERIUM ULCERANS
Detection of M. ulcerans by culture is difficult and may take several weeks at 25°C to 33°C. Chemotherapy plays a role secondary to surgical treatment of indolent, necrotic skin lesions that extend into the derma in infections caused by M. ulcerans (124). However, rifampin may be useful prior to ulceration. Clarithromycin plus rifampin can be a useful adjunct to excision (142) to prevent relapse or other complications (10). In vitro susceptibility testing is inappropriate, but it appears that rifampin resistance is likely to develop with monotherapy (143).
MYCOBACTERIUM HAEMOPHILUM
M. haemophilum is a slowly growing, nonpigmented mycobacterium that requires hemin or ferric ammonium citrate for growth, optimally at 28°C to 30°C. Early reports on the in vitro susceptibility of M. haemophilum were inconsistent, and the role of chemotherapy in the treatment of M. haemophilum infections was unclear. Woods and Washington (124) concluded that this species is resistant to INH, streptomycin, and ethambutol but susceptible to rifampin and/or PAS. M. haemophilum emerged as an important cause of disseminated skin infections in immunocompromised patients, including renal transplant, lymphoma, and AIDS patients (144). The organism also causes disease in immunocompetent hosts, where it causes mild, self-limited, skin infections (145). Correlations have been established between susceptibility test results and clinical efficacy, although virtually all treatment regimens examined included combinations of agents (146). Wild-type isolates of M. haemophilum appear to be susceptible to amikacin, quinolones, rifamycins, clarithromycin, and azithromycin and resistant to PZA and ethambutol and are likely to be resistant to INH and streptomycin (144,146). Surgical excision is usually sufficient for immunocompetent patients (10).
MYCOBACTERIUM GORDONAE
M. gordonae is commonly found in the environment and is readily isolated from water supplies and ice machines. Wayne and Sramek (103) pointed out that, because M. gordonae is so common (30% of nearly 20,000 nontuberculous mycobacteria studied) and disease is rare and cases of M. gordonae infection with clear and compelling clinical correlations are difficult, if not impossible, to find, the pathogenic potential of this species must be extremely low, even in patients with AIDS (147). Nevertheless, it is not uncommon for clinical mycobacteriology laboratories to receive requests for susceptibility testing of M. gordonae isolates. In response to such requests, one could pose the questions offered by Wayne and Sramek (103): (a) Is the isolate truly M. gordonae? (b) Is there convincing evidence that the isolate is playing a role in the disease? In the vast majority of cases, susceptibility testing is inappropriate and may only further mislead the clinician as to the true cause of the disease. The rare occurrence of true M. gordonae infection in patients with AIDS makes this decision more difficult. Mycobacterium interjectum emerged as a potential pathogen and it has been confused with both M. gordonae and Mycobacterium scrofulaceum (148,149). Drug susceptibility testing is not recommended. The ATS/IDSA suggest that ethambutol, rifabutin, clarithromycin, linezolid, and fluoroquinolones be considered in formulating a combination regimen for infections with clear and compelling evidence of cause by M. gordonae (10).
ANTIMYCOBACTERIAL AGENTS: MODES OF ACTION AND MECHANISMS OF RESISTANCE
Isoniazid
The inhibitory activity of INH (isonicotinic acid hydrazide) against M. tuberculosis is remarkably specific and potent. Indeed, no other single antimycobacterial agent has proved to be as active against the M. tuberculosis complex, with such comparatively low toxicity, as INH. However, the activity of INH is less for other species of mycobacteria, and the drug has little or no role in the treatment of certain types of infections, notably, disease caused by RGM and disseminated M. avium infection. INH has no activity against non–acid-fast bacteria and eukaryotic cells. The activity against M. tuberculosis is bactericidal for dividing bacilli but does not kill bacilli in stationary phase or bacilli growing under anaerobic conditions (150–152). However, it is important to note that based on extensive clinical trials, INH is an effective therapeutic and prophylactic agent for clinical latent TB (153). The effect of INH is irreversible within only a few hours of exposure of tubercle bacilli to the drug. INH can bind irreversibly to protein, which is an important interfering factor in the measurement of INH concentrations in biologic fluids.
Isoniazid Activation
INH is a prodrug that the target organism must gratuitously activate in order for it to exert an antibacterial effect. Indeed, the relative inability of most bacteria to activate INH is the primary underlying reason for the selective action of this agent against the M. tuberculosis complex.
The mechanism of INH activation is now reasonably well understood. INH is converted in the presence of nicotinamide adenine dinucleotide (NAD) to an INH-NAD adduct by a catalase-peroxidase encoded by the katG gene (Fig. 5.5A). This process is enhanced by presence of manganese ions, probably involving a shift in redox state from Mn2+ to Mn3+ and back (154). In fact, activation of INH can occur in an enzyme-free system in the presence of Mn3+ ions and nicotinamide coenzymes (155). The derivative generated by this oxidation is either an isonicotinic acyl radical or anion; evidence supporting the production of the free acyl radical (as well as the hydrazyl, peroxo, and pyridyl radical) was reported by Wengenack and Rusnak (156). The oxidized INH forms a covalent link to the carbon at position 4 of the nicotinamide moiety of nicotinamide adenine dinucleotide (hydrogen) (NAD[H]). The kinetics of INH activation and InhA inhibition suggests that the INH-NAD(H) adducts form outside of InhA and then compete with NAD(H) for the InhA binding site (157).
A possible structure of the active form of INH (Fig. 5.5A) was proposed from the X-ray crystal structure of the primary drug target, InhA, an enoyl-acyl carrier protein (ACP) reductase (158). However, it is possible that there is more than one active form of INH. Nguyen et al. (155) isolated another INH-NAD(H) adduct with significant inhibitory activity for InhA (Fig. 5.5B).
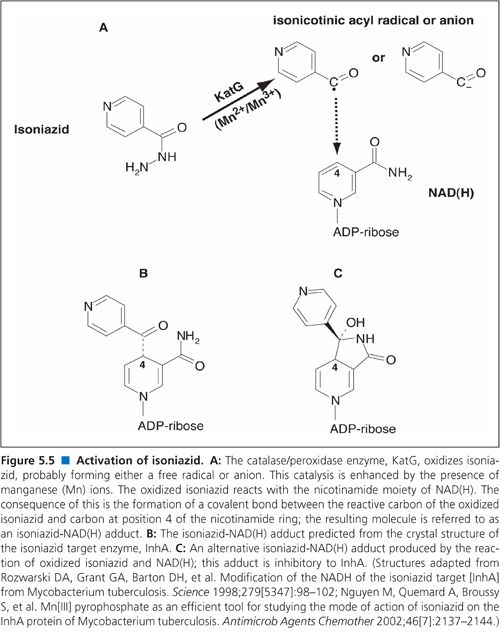
Isoniazid Mechanism of Action
The first insights into the mechanism of action of INH were made by studying the sequence of events that occur following exposure of mycobacteria to this drug. Within 15 minutes of exposure to INH, radioactively labeled drug is taken into the cells and there is a decrease in the ratio of NAD to protein and inhibition of mycolic acid synthesis (159). Within 30 minutes, there is noticeable production of yellow pigment (peroxidase product), and by 60 to 90 minutes, there is a decline in cell viability (159). The bactericidal activity of INH is decreased in growth media depleted of trace metals, and the lethal action of INH appears to be suppressed under anaerobic conditions (159).
Over two decades ago, the demonstration that INH exposure leads to the inhibition of mycolic acid synthesis led to the hypothesis that this is the major cause of mycobacterial cell death. Winder (160) summarized the effects of INH as follows: (a) cells become more fragile and cellular material, including polysaccharides normally acylated to mycolic acids, leaks into the growth medium; (b) intracellular viscosity increases, perhaps due to an increase in cell volume or accumulation of cell wall precursors; (c) cell hydrophobicity decreases; and (d) cells lose the property of acid-fastness. Takayama et al. (161) demonstrated that INH did indeed inhibit mycolic acid synthesis in M. tuberculosis, leading to the accumulation of saturated C26 fatty acids. Central to the process of mycolic acids synthesis are the fatty acid synthesis (FAS) I and II enzyme systems.
The FAS I enzyme system synthesizes saturated fatty acid chains of 16 and 24 carbon atoms. Interestingly, the FAS I system involves a single, multisubunit protein. The FAS II system modifies the C16:0 and C24:0 FAS I products, leading to the formation of mycolic acid chains of up to C56. Unlike FAS I, the FAS II system involves a series of independent enzymes. The range of structures of the α-mycolic acids produced vary between species; consequently, mycolic acid profiling (by high-performance liquid chromatography [HPLC]) can be used for speciating mycobacteria.
One of the FAS II enzymes, the enoyl-ACP reductase or InhA, is the primary target for activated INH (162). The enoyl-ACP reductase catalyzes the saturation of terminal C=C double bond of the growing lipid chain prior to chain elongation by the β-ketoacyl-ACP synthases, KasA and KasB, and subsequent recycling by the β-ketoacyl-ACP reductase (MabA) and β-hydroxyacyl-ACP dehydrase (Fig. 5.6).
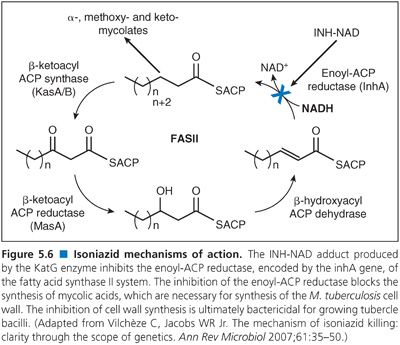
In summary, although there has been controversy regarding the primary target for the INH-NAD adduct, the existing biochemical and genetic evidence indicates that InhA is the primary and most physiologically relevant target for activated INH.
Isoniazid Resistance
The activity of INH is entirely dependent on activation of the prodrug, binding of the INH-NAD adduct to the target, and exerting an inhibitory effect on that target. Thus, changes at any of these steps may lead to a change in susceptibility to INH.
Loss of Isoniazid Activation. An early observation of INH-resistant clinical isolates of M. tuberculosis was the changes in the catalase-peroxidase system of a significant proportion of these organisms (163), and these changes were mapped subsequently to the katG gene (hydroperoxidase I) (164). Such changes in the katG gene (2223 bp) prevent or reduce activation of INH. Indeed, high level INH resistance (MIC >5 μg/mL) correlates with the complete loss of catalase-peroxidase activity while low-level INH resistance (MIC <1 μg/ mL) isolates often retain catalase-peroxidase activity. The changes in the katG gene range from point mutations to small deletions, through to the loss of most or all of the gene (165), although the latter case is rare. The frequency of INH resistance is high (1 in 105 or 106 tubercle bacilli) compared with the other first-line antimycobacterial agents. Point mutations are the most common genetic cause for INH resistance and more than 130 different mutations in katG have been reported that result in amino acid changes and changes in the MIC for INH ranging from 0.2 to 256 μg/mL. The most common mutation in katG is at codon 315 (S315T) accounting for 50% to 95% of INH resistance in clinical isolates (166).
The presence of a nonfunctional katG gene increases susceptibility of M. tuberculosis to oxidative damage. Consequently, in such organisms, compensatory mutations in the regulatory region of the ahpC gene may be present (167,168). However, increased expression of ahpC plays little or no direct role in INH resistance (167).
Altered or Overexpressed inhA Protein. Although mutations in katG account for the majority of INH resistance in M. tuberculosis, mutations in other genes must be involved in approximately 30% of INH-resistant isolates. Several studies have shown that resistance to INH also can be associated with mutations within the inhA gene (S94A) (38,162,169–172). Such mutations appear to alter the hydrogen bonding within the NAD(H) binding site, explaining the reduced affinity of NADH for the inhA protein. Such mutations confer resistance to both INH and ethionamide (173). However, mutations within the inhA gene of the M. tuberculosis complex appear to cause comparatively low-level INH resistance (174,175). Furthermore, the most common inhA-associated mutations in clinical isolates of M. tuberculosis were found to be in the promoter region of the inhA operon (165). In M. tuberculosis, the inhA gene is expressed as an operon with its upstream partner, mab; the Mab protein is a 3-ketoacyl reductase involved in mycolic acid synthesis but not in INH resistance (176). Overall, inhA mutations are rarely the cause of INH resistance and usually occur concurrently with a katG and/or mutation. Indeed, the second most common mutation that causes INH resistance is a mutation in the inhA promoter region (C-15T). The result is an overexpression of inhA messenger RNA (mRNA) and InhA protein (172,177,178).
Other Mechanisms of Isoniazid Resistance. Mutations in kasA, ndh, and glf have been linked with increased resistance to INH (179–182). Furthermore, overexpression of the arylamine N-acetyltransferase (NAT) encoded by the nhoA gene may inactivate INH and cause low-level INH resistance (183,184). However, multiple nucleotide polymorphisms appear to be common in all the genes that have been associated with INH resistance (185), including katG, inhA, kasA and ndh. There is evidence that mycobacteria may have an efflux pump (efpA) that can transport INH out of the cell (186,187) and INH appears to induce increased expression and synthesis of this efflux protein (188).
In summary, although it appears there are several mechanisms of INH resistance and a genetic basis has been established for many of these mechanisms, the predominant cause of INH resistance is associated with mutations in the katG gene, which results in the loss or decreased expression of catalase-peroxidase that is necessary to activate INH.
Rifamycins
The rifamycins (e.g., rifampin, rifapentine, rifabutin, rifaximin, and rifalazil) are potent inhibitors of prokaryotic DNA-dependent RNA polymerases (189), with little activity against the equivalent mammalian enzymes. However, only rifampin (Rifadin, Rimactane, Rifampicin, etc.) and rifapentine (Priftin) are approved (United States) for the treatment of TB. Rifabutin (Mycobutin) is only approved for the treatment/prophylaxis of disseminated MAC disease. Rifalazil (Kaneka Corporation, Osaka, Japan), a benzoxazinorifamycin, is significantly more active against M. tuberculosis compared with other rifamycins, but development of rifalazil for treating TB was discontinued because of an adverse drug effect (viz., flu-like symptom). Rifamixin is only approved for the treatment of enteropathogenic Escherichia coli. The rifamycins are composed of aromatic rings linked by an aliphatic bridge, more specifically an ansa polyhydroxylated bridge connecting two naphthoquinones or naphthhydroquinones (190). Most likely, the lipophilic properties of these molecules aid in the penetration of the drug across the mycobacterial cell wall and are important for binding of the drugs to the target RNA polymerase.
The susceptibility of mycobacteria to rifampin is well documented, and the drug is a first-line component of anti-TB therapy, including DOTS and DOTS-plus (191–193). However, there is significant variation in susceptibility to rifampin between MAC isolates, with the majority being intrinsically resistant. Despite this, the DNA-dependent RNA polymerases isolated from M. intracellulare and M. avium are to be sensitive to rifampin (194,195). Furthermore, substances believed to increase the permeability of the mycobacterial cell wall, such as Tween 80, also cause a significant increase in susceptibility to rifampin. Thus, it appears that the impermeability of the cell wall results in the intrinsic resistance to rifamycins among the mycobacteria where it occurs.
Rifapentine was approved in the United States in 1998 for use against M. tuberculosis (196). In vitro, rifapentine is more active than rifampin, and its metabolite, 25-O-desacetylrifapentine, has equivalent activity to rifampin (197,198). Rifapentine has a significantly longer half-life than rifampin—13.2 to 14.1 hours versus 1.5 to 5 hours, respectively (199–202). Consequently, rifapentine-containing anti-TB regimens are focused on a reduced dosing regimen compared with rifampin, for instance, twice-weekly dosing during the induction phase of therapy (i.e., the first 2 months) and once-weekly dosing during the continuation phase (203). Randomized controlled trials showed that a combination of INH and rifapentine administered weekly for 12 weeks as directly observed therapy (DOT) is as effective for preventing TB as other regimens and is more likely to be completed than a regimen of 9 months of INH daily without DOT (204). An open-label, multicenter, phase III clinical trial was designed to compare the effectiveness and tolerability of a 3-month (12-dose) regimen of weekly rifapentine and INH to the effectiveness of a 9-month regimen of daily INH to prevent TB among high-risk tuberculin skin test reactors, including children and HIV-infected persons, who require treatment of latent TB infection (205). Rifapentine is a weaker inducer of the cytochrome P450 CYP3A system than rifampin (200) and, thus, rifapentine interferes less with drugs metabolized by this system (e.g., protease inhibitors).
Rifabutin has potent activity in vitro against MAC (206) and has equivalent activity as rifapentine against M. tuberculosis (198). However, rifabutin failed to demonstrate efficacy in the treatment of MAC disease in some uncontrolled trials but yielded moderate benefit in other trials (207,208). Rifabutin has been shown to reduce the incidence of MAC disease in HIV-infected patients when used as a prophylactic agent, and the drug has been approved for this use (209,210). Although the basis of the prophylactic activity of rifabutin is not known, this drug has been shown to inhibit the binding of MAC to HT-29 human intestinal carcinoma cells in vitro (211). Thus, rifabutin may prevent colonization of the gastrointestinal tract, which is believed to be a major portal of entry for disseminated MAC disease. Compared with rifampin, rifabutin also has a longer half-life in vivo and is concentrated in tissues, especially the lungs, where levels can be 10 times higher than in serum.
The target site of rifamycins in all bacteria that have been studied is the β-subunit of the prokaryotic RNA polymerase. The β-subunit is one of the five subunits that comprise the polymerase and includes the catalytic center of the enzyme. Rifampin (and presumably the other rifamycins) does not bind in the catalytic center of the β-subunit but at a site upstream from the catalytic center. Thus, rifampin acts as a “plug” rather than a direct catalytic inhibitor and physically blocks (by steric occlusion) de novo RNA from elongating out of the RNA polymerase complex (Fig. 5.7). In this respect, rifamycins have similarity to macrolides (see the following discussion).
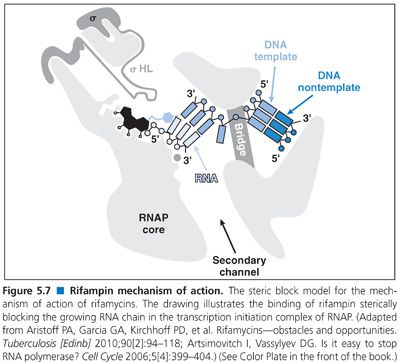
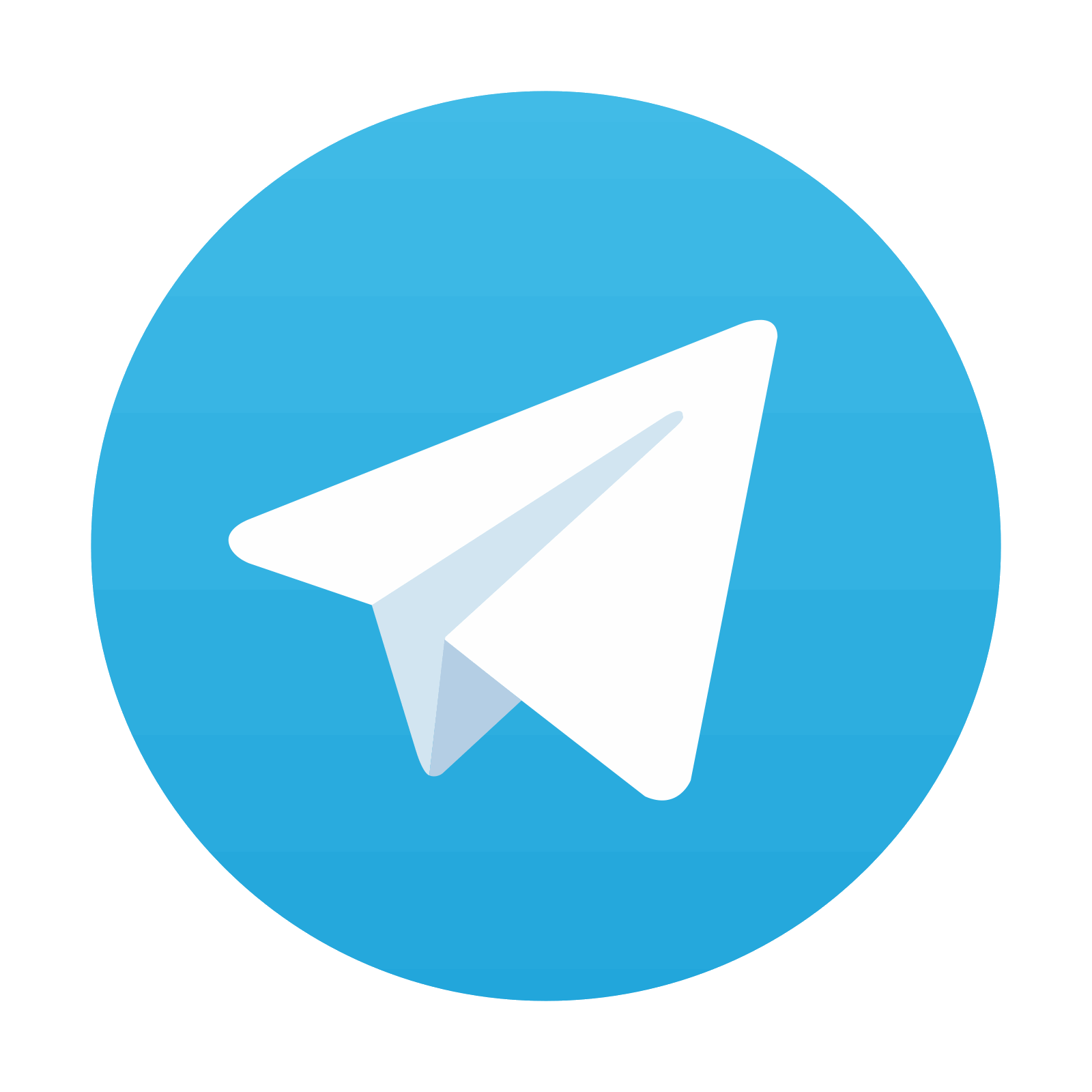
Stay updated, free articles. Join our Telegram channel
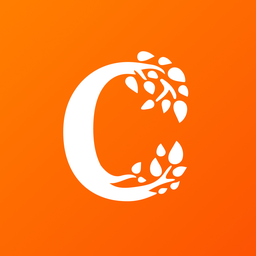
Full access? Get Clinical Tree
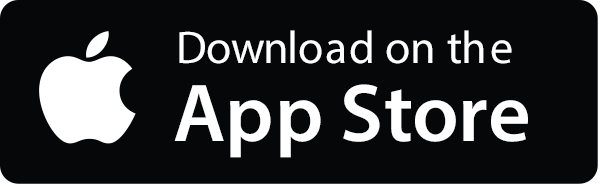
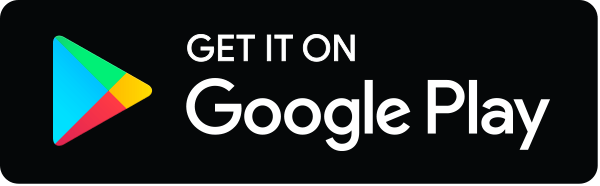