SA Node
Under normal circumstances, the SA node serves as the pacemaker for the heart. Pacemaker activity results from spontaneous phase 4 depolarization (see later). Because cells of the sinus node usually discharge faster than other cells that display automaticity, the SA node normally dominates all other potential pacemakers.
After the SA node discharges, impulses spread rapidly through the atria along the internodal pathways. This rapid conduction allows the atria to contract in unison.
AV Node
Impulses originating in the atria must travel through the AV node to reach the ventricles. In the healthy heart, impulses arriving at the AV node are delayed before going on to excite the ventricles. This delay provides time for blood to fill the ventricles before ventricular contraction.
His-Purkinje System
The fibers of the His-Purkinje system consist of specialized conducting tissue. The function of these fibers is to conduct electrical excitation very rapidly to all parts of the ventricles. Stimulation of the His-Purkinje system is caused by impulses leaving the AV node. These impulses are conducted rapidly down the bundle of His, enter the right and left bundle branches, and then distribute to the many fine branches of the Purkinje fibers (see Fig. 41.1). Because impulses travel quickly through this system, all regions of the ventricles are stimulated almost simultaneously, producing synchronized ventricular contraction with resultant forceful ejection of blood.
Cardiac Action Potentials
Cardiac cells can initiate and conduct action potentials, consisting of self-propagating waves of depolarization followed by repolarization. As in neurons, cardiac action potentials are generated by the movement of ions into and out of cells. These ion fluxes take place by way of specific channels in the cell membrane. In the resting cardiac cell, negatively charged ions cover the inner surface of the cell membrane, whereas positively charged ions cover the external surface. Because of this separation of charge, the cell membrane is said to be polarized. Under proper conditions, channels in the cell membrane open, allowing positively charged ions to rush in. This influx eliminates the charge difference across the cell membrane, and thus the cell is said to depolarize. After depolarization, positively charged ions are extruded from the cell, causing the cell to return to its original polarized state.
In the heart, two kinds of action potentials occur: fast potentials and slow potentials. These potentials differ with respect to the mechanisms by which they are generated, the kinds of cells in which they occur, and the drugs to which they respond.
Profiles of fast and slow potentials are depicted in Fig. 41.2. Please note that action potentials in this figure represent the electrical activity of single cardiac cells. Such single-cell recordings, which are made using experimental preparations, should not be confused with the ECG, which is made using surface electrodes and thus reflects the electrical activity of the entire heart.
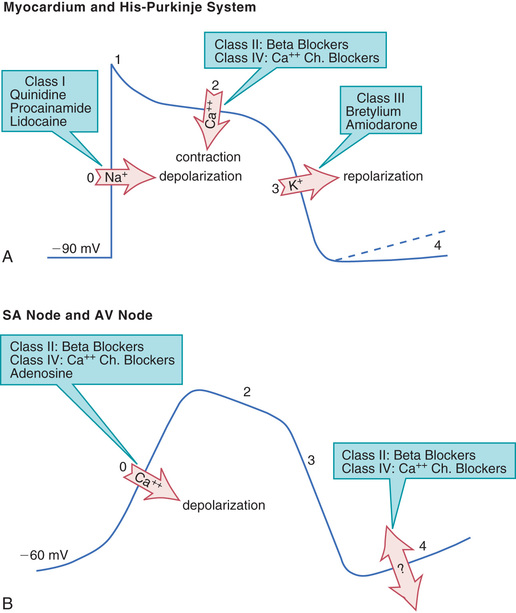
Fast Potentials
Fast potentials occur in fibers of the His-Purkinje system and in atrial and ventricular muscle. These responses serve to conduct electrical impulses rapidly throughout the heart.
As shown in Fig. 41.2A, fast potentials have five distinct phases, labeled 0, 1, 2, 3, and 4. As we discuss each phase, we focus on its ionic basis and its relationship to the actions of antidysrhythmic drugs.
Phase 0.
In phase 0, the cell undergoes rapid depolarization in response to influx of sodium ions. Phase 0 is important in that the speed of phase 0 depolarization determines the velocity of impulse conduction. Drugs that decrease the rate of phase 0 depolarization (by blocking sodium channels) slow impulse conduction through the His-Purkinje system and myocardium.
Phase 1.
During phase 1, rapid (but partial) repolarization takes place. Phase 1 has no relevance to antidysrhythmic drugs.
Phase 2.
Phase 2 consists of a prolonged plateau in which the membrane potential remains relatively stable. During this phase, calcium enters the cell and promotes contraction of atrial and ventricular muscle. Drugs that reduce calcium entry during phase 2 do not influence cardiac rhythm. However, because calcium influx is required for contraction, these drugs can reduce myocardial contractility.
Phase 3.
In phase 3, rapid repolarization takes place. This repolarization is caused by extrusion of potassium from the cell. Phase 3 is relevant in that delay of repolarization prolongs the action potential duration and thereby prolongs the effective refractory period (ERP). (The ERP is the time during which a cell is unable to respond to excitation and initiate a new action potential. Therefore extending the ERP prolongs the minimal interval between two propagating responses.) Phase 3 repolarization can be delayed by drugs that block potassium channels.
Phase 4.
During phase 4, two types of electrical activity are possible: (1) the membrane potential may remain stable (solid line in Fig. 41.2A) or (2) the membrane may undergo spontaneous depolarization (dashed line). In cells undergoing spontaneous depolarization, the membrane potential gradually rises until a threshold potential is reached. At this point, rapid phase 0 depolarization takes place, setting off a new action potential. Hence it is phase 4 depolarization that gives cardiac cells automaticity (i.e., the ability to initiate an action potential through self-excitation). The capacity for self-excitation makes potential pacemakers of all cells that have it.
Under normal conditions, His-Purkinje cells undergo very slow spontaneous depolarization, and myocardial cells do not undergo any. However, under pathologic conditions, significant phase 4 depolarization may occur in all of these cells, and especially in Purkinje fibers. When this happens, a dysrhythmia can result.
Slow Potentials
Slow potentials occur in cells of the SA node and AV node. The profile of a slow potential is depicted in Fig. 41.2B. Like fast potentials, slow potentials are generated by ion fluxes. However, the specific ions involved are not the same for every phase.
From a physiologic and pharmacologic perspective, slow potentials have three features of special significance: (1) phase 0 depolarization is slow and mediated by calcium influx, (2) these potentials conduct slowly, and (3) spontaneous phase 4 depolarization in the SA node normally determines heart rate.
Phase 0.
Phase 0 (depolarization phase) of slow potentials differs significantly from phase 0 of fast potentials. As we can see from Fig. 41.2, whereas phase 0 of fast potentials is caused by a rapid influx of sodium, phase 0 of slow potentials is caused by slow influx of calcium. Because calcium influx is slow, the rate of depolarization is slow; and because depolarization is slow, these potentials conduct slowly. This explains why impulse conduction through the AV node is delayed. Phase 0 of the slow potential is of therapeutic significance in that drugs that suppress calcium influx during phase 0 can slow (or stop) AV conduction.
Phases 2 and 3.
Slow potentials lack a phase 1 (see Fig. 41.2B). Phases 2 and 3 of the slow potential are not significant with respect to the actions of antidysrhythmic drugs.
Phase 4.
Cells of the SA node and AV node undergo spontaneous phase 4 depolarization. The ionic basis of this phenomenon is complex and incompletely understood.
Under normal conditions, the rate of phase 4 depolarization in cells of the SA node is faster than in all other cells of the heart. As a result, the SA node discharges first and determines heart rate. Hence the SA node is referred to as the cardiac pacemaker.
As shown in Fig. 41.2B, two classes of drugs (beta blockers and calcium channel blockers) can suppress phase 4 depolarization. By doing so, these agents can decrease automaticity in the SA node.
The Electrocardiogram
The ECG provides a graphic representation of cardiac electrical activity. The ECG can be used to identify dysrhythmias and monitor responses to therapy. (Note: In referring to the electrocardiogram, two abbreviations may be used: EKG and ECG.)
The major components of an ECG are shown in Fig. 41.3. As we can see, three features are especially prominent: the P wave, the QRS complex, and the T wave. The P wave is caused by depolarization in the atria. Therefore the P wave corresponds to atrial contraction. The QRS complex is caused by depolarization of the ventricles, so the QRS complex corresponds to ventricular contraction. If conduction through the ventricles is slowed, the QRS complex will widen. The T wave is caused by repolarization of the ventricles, so this wave is not associated with overt physical activity of the heart.
In addition to the features just described, the ECG has three other components of interest: the PR interval, the QT interval, and the ST segment. The PR interval is defined as the time between the onset of the P wave and the onset of the QRS complex. Lengthening of this interval indicates a delay in conduction through the AV node. Several drugs increase the PR interval. The QT interval is defined as the time between the onset of the QRS complex and completion of the T wave. This interval is prolonged by drugs that delay ventricular repolarization. The ST segment is the portion of the ECG that lies between the end of the QRS complex and the beginning of the T wave. Digoxin depresses the ST segment.
Generation of Dysrhythmias
Dysrhythmias arise from two fundamental causes: disturbances of impulse formation (automaticity) and disturbances of impulse conduction. One or both of these disturbances underlie all dysrhythmias. Factors that may alter automaticity or conduction include hypoxia, electrolyte imbalance, cardiac surgery, reduced coronary blood flow, myocardial infarction, and antidysrhythmic drugs.
Disturbances of Automaticity
Disturbances of automaticity can occur in any part of the heart. Cells normally capable of automaticity (cells of the SA node, AV node, and His-Purkinje system) can produce dysrhythmias if their normal rate of discharge changes. In addition, dysrhythmias may be produced if tissues that do not normally express automaticity (atrial and ventricular muscle) develop spontaneous phase 4 depolarization.
Altered automaticity in the SA node can produce tachycardia or bradycardia. Excessive discharge of sympathetic neurons that innervate the SA node can augment automaticity to such a degree that sinus tachycardia results. Excessive vagal (parasympathetic) discharge can suppress automaticity to such a degree that sinus bradycardia results.
Increased automaticity of Purkinje fibers is a common cause of dysrhythmias. The increase can be brought on by injury and by excessive stimulation of Purkinje fibers by the sympathetic nervous system. If Purkinje fibers begin to discharge faster than the SA node, they will escape control by the SA node; potentially serious dysrhythmias can result.
Under special conditions, automaticity may develop in cells of atrial and ventricular muscle. If these cells fire faster than the SA node, dysrhythmias will result.
PATIENT-CENTERED CARE ACROSS THE LIFE SPAN
Antidysrhythmic Drugs
Life Stage | Patient Care Concerns |
Infants | See later entry, “Breastfeeding women.” |
Children/adolescents | Some antidysrhythmic drugs can be used safely in children, just in smaller doses. These include disopyramide, flecainide, and sotalol. Side-effect profiles are similar to those of adults. |
Pregnant women | Many of the drugs discussed in this chapter are classified in U.S. Food and Drug Administration Pregnancy Risk Category C or D. Animal studies show adverse fetal effects, and in Category D, there is evidence of human fetal risk. Benefits should outweigh the risks. Dronedarone is classified in Pregnancy Risk Category X. |
Breastfeeding women | For most of the drugs discussed in this chapter, data are lacking regarding transmission of drug from mother to infant through breast milk. Breastfeeding is contraindicated in women taking dronederone. |
Older adults | Aging alters the absorption, distribution, metabolism, and elimination of antidysrhythmic drugs. Liver and kidney function must be monitored, and antiarrhythmic dosing may need to be adjusted for age. Older-adult patients are also more susceptible to the side effects of many antidysrhythmics, including bradycardia, orthostatic hypotension, urinary retention, and falls. |
Disturbances of Conduction
AV Block
Impaired conduction through the AV node produces varying degrees of AV block. If impulse conduction is delayed (but not prevented entirely), the block is termed first degree. If some impulses pass through the node but others do not, the block is termed second degree. If all traffic through the AV node stops, the block is termed third degree.
Reentry (Recirculating Activation)
Reentry, also referred to as recirculating activation, is a generalized mechanism by which dysrhythmias can be produced. Reentry causes dysrhythmias by establishing a localized, self-sustaining circuit capable of repetitive cardiac stimulation. Reentry results from a unique form of conduction disturbance. The mechanism of reentrant activation and the effects of drugs on this process are described next.
In normal impulse conduction, electrical impulses travel down both branches of the Purkinje fiber to cause excitation of the muscle at two locations (Fig. 41.4A). Impulses created within the muscle travel in both directions (to the right and to the left) away from their sites of origin. Those impulses that are moving toward each other meet midway between the two branches of the Purkinje fiber. Because in the wake of both impulses the muscle is in a refractory state, neither impulse can proceed further, so both impulses stop.
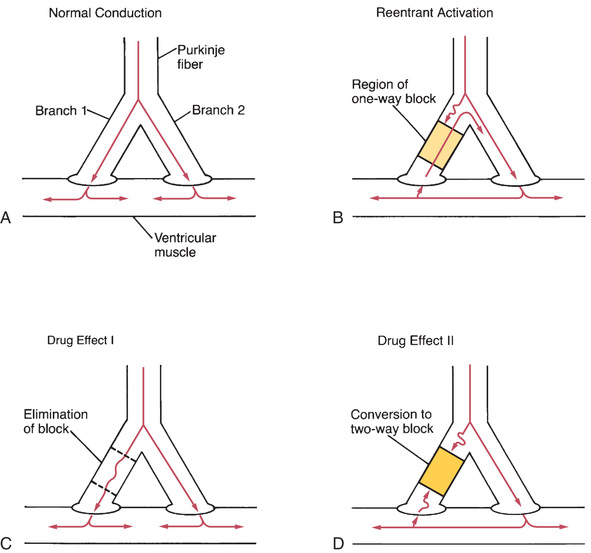
In a reentrant circuit (Fig. 41.4B), there is a region of one-way conduction block of one branch of the Purkinje fiber. This region prevents conduction of impulses downward (toward the muscle), but does not prevent impulses from traveling upward. (Impulses can travel back up the block because impulses in muscle are very strong and hence are able to pass the block, whereas impulses in the Purkinje fiber are weaker and so are unable to pass.) A region of one-way block is essential for reentrant activation.
How does one-way block lead to reentrant activation? As an impulse travels down the Purkinje fiber, it is blocked in one branch but continues unimpeded in the other branch. Upon reaching the tip of the second (unblocked) branch, the impulse stimulates the muscle. As described earlier, the impulse in the muscle travels to the right and to the left away from its site of origin. However, in this new situation, as the impulse travels toward the impaired branch of the Purkinje fiber, it meets no impulse coming from the other direction and continues on, resulting in stimulation of the terminal end of the first (blocked) branch. This stimulation causes an impulse to travel backward up the blocked branch of the Purkinje fiber. Because blockade of conduction in that branch is one way (downward only), the impulse can pass upward through the region of block and then back down into the unblocked branch, causing reentrant activation of this branch. Under proper conditions, the impulse will continue to cycle indefinitely, resulting in repetitive ectopic beats.
There are two mechanisms by which drugs can abolish a reentrant dysrhythmia. First, drugs can improve conduction in the sick branch of the Purkinje fiber, and can thereby eliminate the one-way block (Fig. 41.4C). Alternatively, drugs can suppress conduction in the sick branch, thereby converting one-way block into two-way block (Fig. 41.4D).
Classification of Antidysrhythmic Drugs
According to the Vaughan Williams classification scheme, the antidysrhythmic drugs fall into five groups (Table 41.1). There are four major classes of antidysrhythmic drugs (classes I, II, III, and IV) and a fifth group that includes adenosine and digoxin. Membership in classes I through IV is determined by effects on ion movements during slow and fast potentials (see Fig. 41.2).
TABLE 41.1
Vaughan Williams Classification of Antidysrhythmic Drugs
CLASS I: SODIUM CHANNEL BLOCKERS
Class IA
Quinidine
Procainamide [Procan , generic in United States]
Disopyramide [Norpace, Rythmodan ]
Class IB
Phenytoin [Dilantin]
Mexiletine [Mexitil]
Class IC
Flecainide [Tambocor]
Propafenone [Rythmol, Rythmol SR]
CLASS II: BETA BLOCKERS
Propranolol [Inderal, Inderal LA]
Acebutolol [Sectral]
CLASS III: POTASSIUM CHANNEL BLOCKERS (DRUGS THAT DELAY REPOLARIZATION)
Amiodarone [Cordarone, Pacerone]
Dronedarone [Multaq]
Sotalol [Betapace, Betapace AF]
Dofetilide [Tikosyn]
CLASS IV: CALCIUM CHANNEL BLOCKERS
Verapamil [Calan, Covera -HS, Verelan]
Diltiazem [Cardizem, Dilacor-XR, Tiazac, others]
OTHER ANTIDYSRHYTHMIC DRUGS
Digoxin [Lanoxin]
Class I: Sodium Channel Blockers
Class I drugs block cardiac sodium channels (see Fig. 41.2A). By doing so, these drugs slow impulse conduction in the atria, ventricles, and His-Purkinje system. Class I constitutes the largest group of antidysrhythmic drugs.
Class II: Beta Blockers
Class II consists of beta-adrenergic blocking agents. As suggested by Fig. 41.2, these drugs reduce calcium entry (during fast and slow potentials) and depress phase 4 depolarization (in slow potentials only). Beta blockers have three prominent effects on the heart:
Cardiac effects of the beta blockers are nearly identical to those of the calcium channel blockers.
Class III: Potassium Channel Blockers (Drugs That Delay Repolarization)
Class III drugs block potassium channels (Fig. 41.2A) and thereby delay repolarization of fast potentials. By delaying repolarization, these drugs prolong both the action potential duration and the effective refractory period.
Class IV: Calcium Channel Blockers
Only two calcium channel blockers—verapamil and diltiazem—are employed as antidysrhythmics. As indicated in Fig. 41.2, calcium channel blockade has the same effect on cardiac action potentials as does beta blockade. Accordingly, verapamil, diltiazem, and beta blockers have nearly identical effects on cardiac function—namely, reduction of automaticity in the SA node, delay of conduction through the AV node, and reduction of myocardial contractility. Antidysrhythmic benefits derive from suppressing AV nodal conduction.
Other Antidysrhythmic Drugs
Adenosine and digoxin do not fit into the four major classes of antidysrhythmic drugs. Both drugs suppress dysrhythmias by decreasing conduction through the AV node and reducing automaticity in the SA node.
Prodysrhythmic Effects of Antidysrhythmic Drugs
Virtually all of the drugs used to treat dysrhythmias have prodysrhythmic (proarrhythmic) effects. That is, all of these drugs can worsen existing dysrhythmias and generate new ones. This ability was documented dramatically in the Cardiac Arrhythmia Suppression Trial (CAST), in which use of class IC drugs (encainide and flecainide) to prevent dysrhythmias after myocardial infarction actually doubled the rate of mortality. Because of their prodysrhythmic actions, antidysrhythmic drugs should be used only when dysrhythmias are symptomatically significant, and only when the potential benefits clearly outweigh the risks. Applying this guideline, it would be inappropriate to give antidysrhythmic drugs to a patient with nonsustained ventricular tachycardia because this dysrhythmia does not significantly reduce cardiac output. Conversely, when a patient is facing death from ventricular fibrillation, any therapy that might work must be tried. In this case the risk for prodysrhythmic effects is clearly outweighed by the potential benefits of stopping the fibrillation.
Overview of Common Dysrhythmias and Their Treatment
The common dysrhythmias can be divided into two major groups: supraventricular dysrhythmias and ventricular dysrhythmias. In general, ventricular dysrhythmias are more dangerous than supraventricular dysrhythmias. With either type, intervention is required only if the dysrhythmia interferes with effective ventricular pumping. Treatment often proceeds in two phases: (1) termination of the dysrhythmia (with electrical countershock, drugs, or both), followed by (2) long-term suppression with drugs. Dysrhythmias can also be treated with an implantable cardioverter-defibrillator (ICD) or by destroying small areas of cardiac tissue using radiofrequency (RF) catheter ablation.
It is important to appreciate that drug therapy of dysrhythmias is highly empiric (i.e., based largely on the response of the patient and not on scientific principles). In practice, this means that, even after a dysrhythmia has been identified, we cannot predict with certainty just which drugs will be effective. Frequently, trials with several drugs are required before control of rhythm is achieved. In the discussion that follows, only first-choice drugs are considered.
Supraventricular Dysrhythmias
Supraventricular dysrhythmias are dysrhythmias that arise in areas of the heart above the ventricles (atria, SA node, AV node). Supraventricular dysrhythmias per se are not especially harmful because dysrhythmic activity within the atria does not significantly reduce cardiac output (except in patients with valvular disorders and heart failure [HF]). Supraventricular tachydysrhythmias can be dangerous, however, in that atrial impulses are likely to traverse the AV node, resulting in excitation of the ventricles. If the atria drive the ventricles at an excessive rate, diastolic filling will be incomplete and cardiac output will decline. Hence, when treating supraventricular tachydysrhythmias, the objective is frequently slowing of ventricular rate (by blocking impulse conduction through the AV node) and not elimination of the dysrhythmia itself. Of course, if treatment did abolish the dysrhythmia, this outcome would not be unwelcome. Acute treatment of supraventricular dysrhythmias is accomplished with vagotonic maneuvers, direct-current (DC) cardioversion, and certain drugs: class II agents, class IV agents, adenosine, and digoxin.
Atrial Fibrillation
Atrial fibrillation is the most common sustained dysrhythmia, affecting about 2.6 million people in the United States. The disorder is caused by multiple atrial ectopic foci firing randomly; each focus stimulates a small area of atrial muscle. This chaotic excitation produces a highly irregular atrial rhythm. Depending on the extent of impulse transmission through the AV node, ventricular rate may be very rapid or nearly normal.
In addition to compromising cardiac performance, atrial fibrillation carries a high risk for stroke because, in patients with atrial fibrillation, some blood can become trapped in the atria (rather than flowing straight through to the ventricles), thereby permitting formation of a clot. When normal sinus rhythm is restored, the clot may become dislodged and then may travel to the brain to cause stroke.
Treatment of atrial fibrillation has two goals: improvement of ventricular pumping and prevention of stroke. Pumping can be improved by either (1) restoring normal sinus rhythm or (2) slowing ventricular rate. The preferred method is to slow ventricular rate by long-term therapy with a beta blocker (atenolol or metoprolol) or a cardioselective calcium channel blocker (diltiazem or verapamil), both of which impede conduction through the AV node. For patients who elect to restore normal rhythm, options are DC cardioversion, short-term treatment with drugs (e.g., amiodarone, sotalol), or RF ablation of the dysrhythmia source.
To prevent stroke, patients are treated with warfarin or newer anticoagulants. For those undergoing treatment to restore normal sinus rhythm, warfarin should be taken for 3 weeks before the procedure and for 4 weeks after. For those taking an antidysrhythmic drug long term to control ventricular rate, warfarin must be taken long term too. Alternatives to warfarin include three new oral anticoagulants—apixiban [Eliquis], dabigatran [Pradaxa], and rivaroxaban [Xarelto]—and antiplatelet drugs (either aspirin alone or aspirin plus clopidogrel).
Atrial Flutter
Atrial flutter is caused by an ectopic atrial focus discharging at a rate of 250 to 350 times a minute. Ventricular rate is considerably slower, however, because the AV node is unable to transmit impulses at this high rate. Typically, one atrial impulse out of two reaches the ventricles. The treatment of choice is DC cardioversion, which almost always converts atrial flutter to normal sinus rhythm. Cardioversion may also be achieved with intravenous (IV) ibutilide. To prevent the dysrhythmia from recurring, patients may need long-term therapy with drugs—either a class IC agent (flecainide or propafenone) or a class III agent (amiodarone, dronedarone, sotalol, dofetilide).
There are two alternatives to cardioversion: (1) RF ablation of the dysrhythmia focus and (2) control of ventricular rate with drugs. As with atrial fibrillation, ventricular rate is controlled with drugs that suppress AV conduction: verapamil, diltiazem, or a beta blocker.
Like atrial fibrillation, atrial flutter poses a risk for stroke, which can be reduced by treatment with anticoagulants.
Sustained Supraventricular Tachycardia (SVT)
This is usually caused by an AV nodal reentrant circuit. Heart rate is increased to 150 to 250 beats/minute. SVT often responds to interventions that increase vagal tone, such as carotid sinus massage or the Valsalva maneuver. If these are ineffective, an IV beta blocker or calcium channel blocker can be tried. With these drugs, ventricular rate will be slowed even if the dysrhythmia persists. When the dysrhythmia has been controlled, beta blockers and/or calcium channel blockers can be taken orally to prevent recurrence. As a last resort, amiodarone can be used for prevention.
Ventricular Dysrhythmias
In contrast to atrial dysrhythmias, which are generally benign, ventricular dysrhythmias can cause significant disruption of cardiac pumping. Accordingly, the usual objective is to abolish the dysrhythmia. Cardioversion is often the treatment of choice. When antidysrhythmic drugs are indicated, agents in class I or class III are usually employed.
SVT
Ventricular tachycardia arises from a single, rapidly firing ventricular ectopic focus, typically located at the border of an old infarction. The focus drives the ventricles at a rate of 150 to 250 beats/minute. Because the ventricles cannot pump effectively at these rates, immediate intervention is required. Cardioversion is the treatment of choice. If cardioversion fails to normalize rhythm, IV amiodarone should be administered; lidocaine and procainamide are alternatives. For long-term management, drugs (e.g., sotalol, amiodarone) or an ICD may be employed.
Ventricular Fibrillation
Ventricular fibrillation is a life-threatening emergency that requires immediate treatment. This dysrhythmia results from the asynchronous discharge of multiple ventricular ectopic foci. Because many different foci are firing, and because each focus initiates contraction in its immediate vicinity, localized twitching takes place all over the ventricles, making coordinated ventricular contraction impossible. As a result, the pumping action of the heart stops. In the absence of blood flow, the patient becomes unconscious and cyanotic. If heartbeat is not restored rapidly, death soon follows. Electrical countershock (defibrillation) is applied to eliminate fibrillation and restore cardiac function. If necessary, IV lidocaine can be used to enhance the effects of defibrillation. Procainamide may also be helpful. Amiodarone can be used for long-term suppression. As an alternative, an ICD may be employed.
Premature Ventricular Complex (PVC)
PVCs are beats that occur before they should in the cardiac cycle. These beats are caused by ectopic ventricular foci. PVCs may arise from a single ectopic focus or from several foci. In the absence of additional signs of heart disease, PVCs are benign and not usually treated. However, in the presence of acute myocardial infarction, PVCs may predispose the patient to ventricular fibrillation. In this case therapy is required. A beta blocker is the agent of choice.
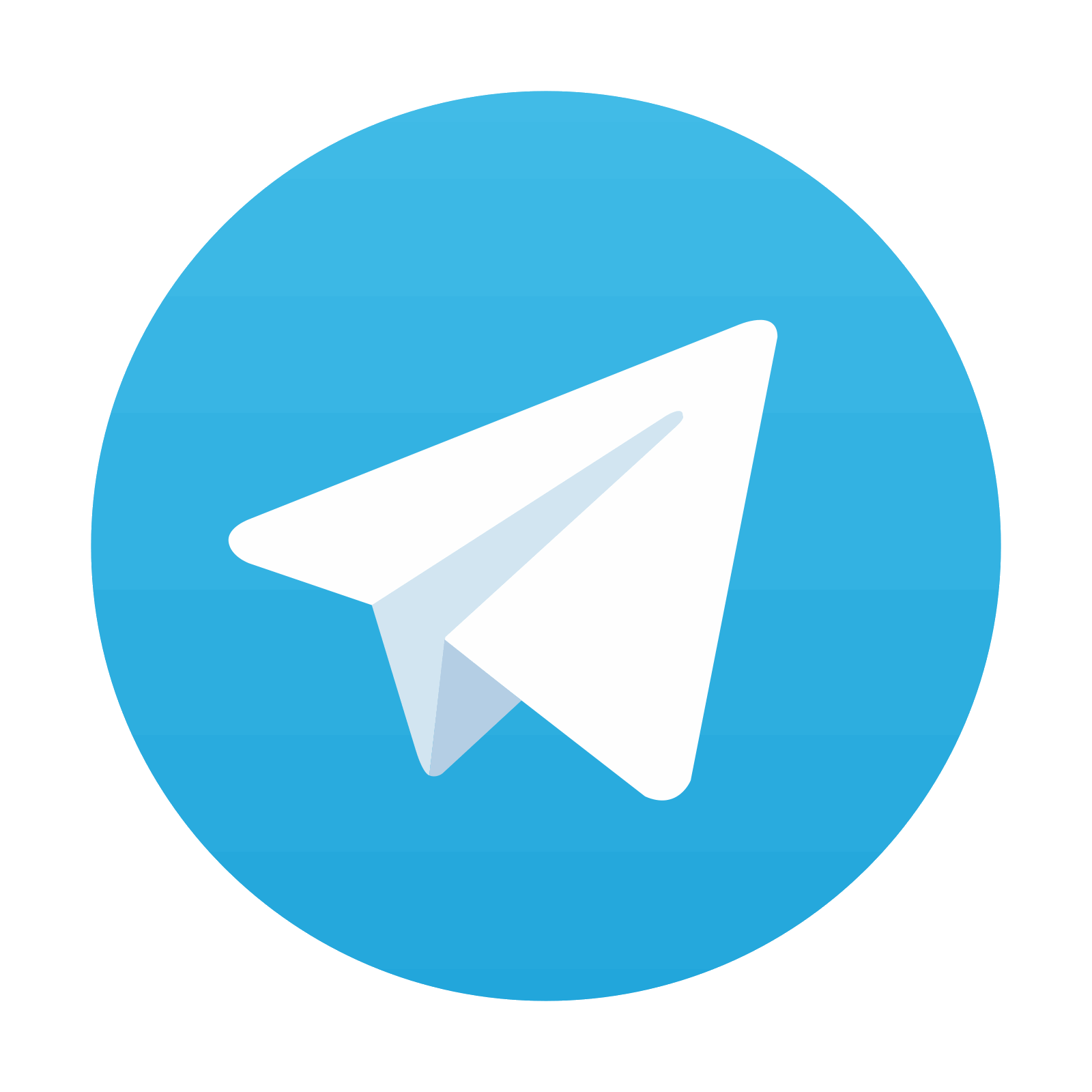
Stay updated, free articles. Join our Telegram channel
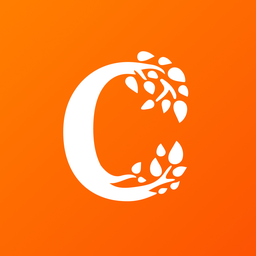
Full access? Get Clinical Tree
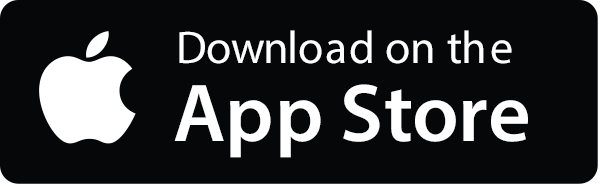
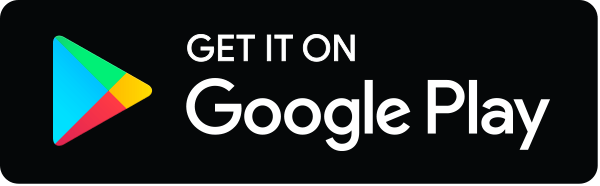