Alterations in Blood Flow
Teresa Grigsby Loftsgaarden
Key Questions
• What is the relationship among vessel resistance, blood pressure, and blood flow?
• What are the determinants of transcapillary exchange of fluids, electrolytes, and nutrients?
• How do arterial and venous obstructions develop?
• What are the clinical consequences of acute and chronic arterial obstruction?
• What are the clinical consequences of superficial and deep venous obstructions?
http://evolve.elsevier.com/Copstead/
The primary functions of the circulatory system are the transportation of oxygen and nutrients and the removal of metabolic waste products within the body. To perform these functions, a complex circuitry of vessels traverses the body (Figure 15-1), powered by the pumping action of the heart. Propulsion of blood through the lungs is provided by the right ventricle, whereas systemic blood flow is driven by the left ventricle.
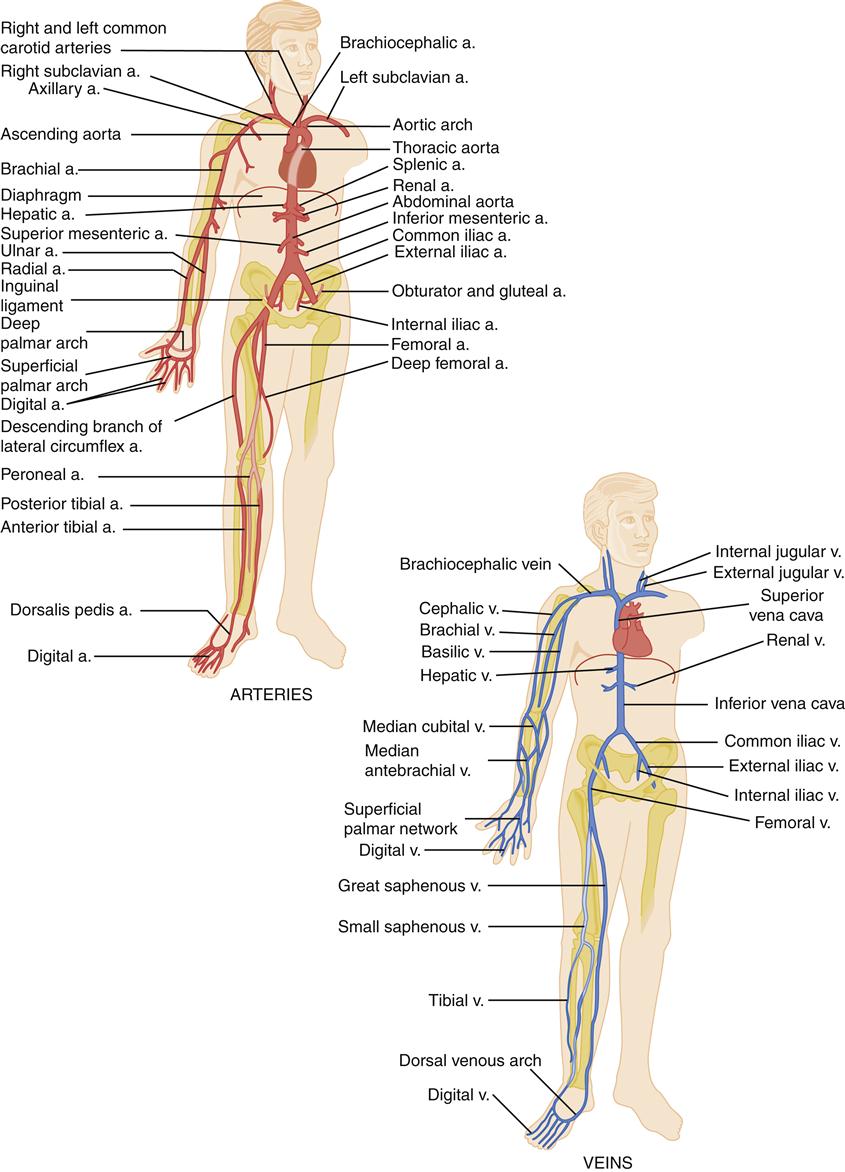
a., Artery; v., vein. (From Black JM, Hawks J: Medical-surgical nursing: clinical management for positive outcomes, ed 8, Philadelphia, 2008, Saunders, p 1273.)
Nutrients are absorbed into the blood as it moves through the gastrointestinal tract via the splanchnic circulation. Oxygen uptake and the release of carbon dioxide occur in the specialized vascular bed of the pulmonary circulation. The liver, with its extensive blood supply, has a major role in metabolism and generation of metabolic waste products. These, and other metabolic by-products, are carried by the blood to the kidneys for elimination. Inadequate circulation in the lungs, liver, or kidneys may interfere with the removal of metabolic wastes from the body. Effective transportation of oxygen and nutrients and removal of waste materials depend on proper functioning of the circulatory system.
Aging produces significant changes in the circulatory system, altering the ability of the system to carry out its functions and increasing susceptibility to certain disease processes. The effects of the aging process on the circulatory system are summarized in Geriatric Considerations: Changes in the Circulatory System.
Organization of the Circulatory and Lymphatic Systems
After passing through the pulmonary circulatory system and leaving the left ventricle, blood flows through a graduated series of tubes to tissues of the body before returning to the right side of the heart. The powerful left ventricle propels the blood to the aorta, arteries, arterioles, and, finally, to the capillary beds. Here the proximity of capillary endothelium to the other cells of the body facilitates movement of nutrients and oxygen into the cells and removal of cellular metabolic wastes. Capillary blood is then collected by venules, which flow into veins, returning blood to the venae cavae and the right side of the heart (Figure 15-2). The complete process, moving approximately 5 L of blood through the entire circuit, takes only about 1 minute.
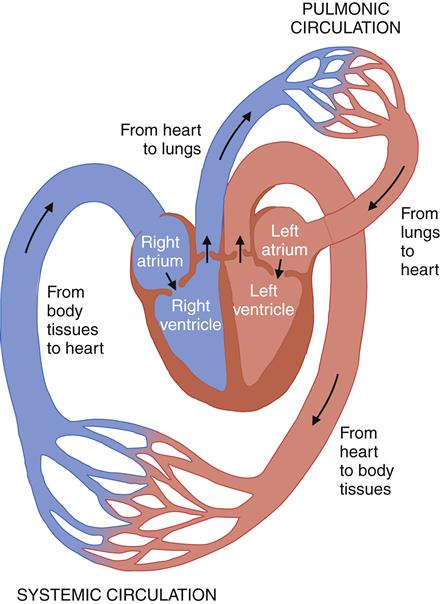
Beginning from the body tissues, blood returns to the right side of the heart, through the right atria to the right ventricle, which propels it into the lungs. In the lungs, the metabolic waste carbon dioxide is removed and oxygen is replenished. Oxygenated blood leaves the pulmonic circulation and returns to the heart via the left atrium and then to the left ventricle. From the left side of the heart, the oxygenated blood enters the systemic circulation, where oxygen is delivered to the tissues in exchange for metabolic wastes. (From Black JM, Hawks J: Medical-surgical nursing: clinical management for positive outcomes, ed 8, Philadelphia, 2008, Saunders, p 1344.)
The lymphatic circulation is a specialized system of channels and tissues (nodes). It is not arranged in a circuit, as is the vascular system. Instead, the lymphatic vessels begin blindly, deep in the connective tissue. One of the functions of the lymphatic system is to reabsorb fluid that leaks out of the vascular network into the interstitium and return it to the general circulation. During the process of cellular exchange within the capillary bed, some fluid moves into the interstitium and fails to return to the vascular bed. This lost fluid can amount to as much as 2 to 4 L/day. At this circulatory level, lymphatic vessels lie in close proximity to the capillary vasculature. The fluid, now called lymph, is absorbed by the lymphatic vessels and returned to the venous circulation by way of the thoracic duct and the right lymphatic duct (Figure 15-3). Lymphatic drainage of the breast is illustrated in Figure 15-4.
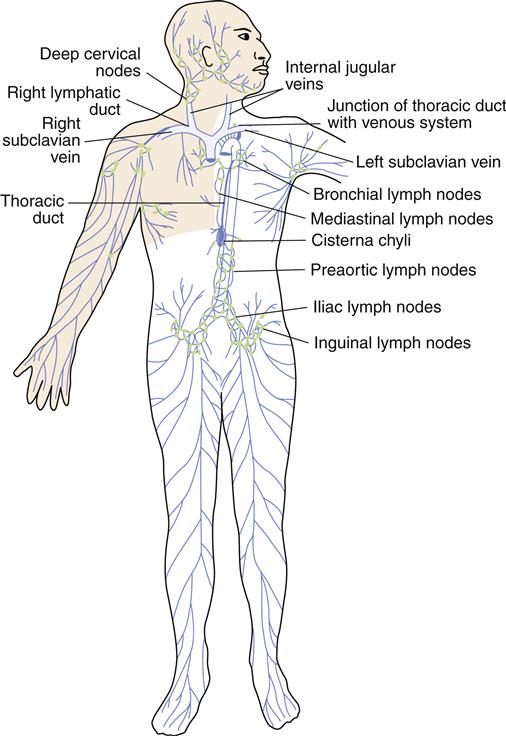
Lymphatic capillaries collect the excess fluid from the vascular capillaries, returning it to the venous circulation at the junction of the internal jugular and subclavian veins. (From Monahan FD et al: Phipps’ medical-surgical nursing: health and illness perspectives, ed 8, Philadelphia, 2007, Mosby, p 936.)
Vessel Structure
To perform their specialized functions, the blood and lymphatic vessels are different in their structure. Knowledge of the morphology of these vessels is essential for an understanding of the alterations in function produced by disease.
The primary differences between the smaller arterial and venous vessels are in terms of the quantities of muscle and connective tissue present. In arterioles, the principal tissue is smooth muscle, whereas in venules, smooth muscle is scarce and connective tissue dominates. The composition of the walls and the size and shape of the vessels also vary in larger arteries and veins. Capillary walls are composed of a single layer of endothelial cells. These simple structures carry out extraordinarily complex functions.
Anatomy of Arteries and Veins
The walls of both arteries and veins are composed of three microscopically distinct layers, or tunicae: the intima, the media, and the adventitia. The histologic constituents of these tunicae are similar in arteries and veins (Figure 15-5). Generally, the walls of veins are not as thick as the walls of arteries, but the lumina are larger.
The intima consists of a layer of endothelial cells that is in direct contact with the blood as it flows through the vessel. Periodically, the intimal layer of veins protrudes into the lumen, creating valves that prevent the backflow of blood. Arterial intima is characterized by an inner elastic membrane next to the endothelial cells. This elastic membrane is thickest in the aorta and decreases in density until only scattered elastic fibers can be identified in the smallest arterioles. With increasing age, the intimal arterial wall becomes thicker and less elastic. This interferes with diffusion of nutrients into the wall, causing the internal elastic membrane to degenerate and calcify.
The media, or middle layer, exhibits the greatest difference between arteries and veins. In arteries the media is the thickest of all the tunicae. Large arteries have smooth muscle fibers arranged in a circular pattern and interspersed with elastic fibers. Progressing from arteries to ever-smaller arterioles, the smooth muscle remains but the elastic tissue disappears. This thick, smooth muscle layer is responsible for the firmness and limited distensibility of arterial vessels. With advancing age, changes in the intima result in decreased nutrition reaching the media, causing degeneration of the smooth muscle tissue. In veins, the media also has smooth muscle, usually arranged in a circular pattern with some longitudinal strands. The quantity of smooth muscle decreases as the veins become larger. Venous media also contains collagenous connective tissue, but elastic tissue is rare except in the largest veins.
In veins, the adventitia is the thickest of the tunicae. It is composed of collagenous connective tissue and longitudinal smooth muscle. In larger arteries there is a discernible external elastic membrane in the adventitia. This membrane disappears as the arteries decrease in diameter. Arterial adventitia consists predominantly of collagenous
connective tissue. Some larger vessels also contain isolated, longitudinally arranged fibers of smooth muscle.
Anatomy of Capillaries
Capillaries are composed of a single thickness of endothelial cells attached to a protein network called the basement membrane. Moving from the end of an arteriole to the beginning of a venule, capillaries narrow to a diameter barely sufficient for a single red blood cell (RBC) to pass through the aperture. In some tissues, one or two smooth muscle cells form a precapillary sphincter that controls flow through the vessel (Figure 15-6).
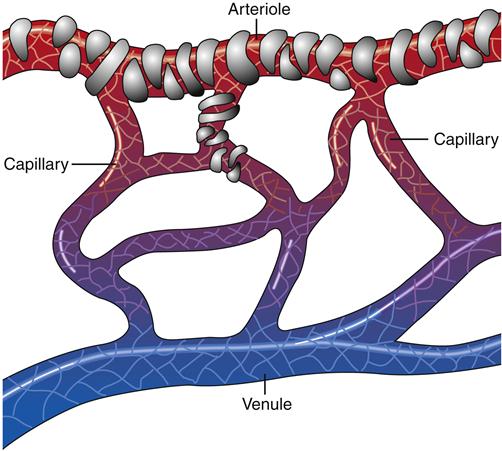
There are spaces between the endothelial cells that vary in size among organ systems. These spaces, or pores, permit certain constituents to pass in and out of the capillaries. For example, capillary beds in the brain have little or no spaces and permit the passage of only certain molecules. The space between endothelial cells of the brain is so small that it is referred to as the blood-brain barrier. In parts of the kidneys, however, capillaries are more porous, allowing much larger molecules to move between the circulation and the filtrate (urine). The size of these spaces determines the capillary permeability of a specific capillary bed.
Lymphatic Structure
Lymphatic vessels are thin walled and most resemble veins in their appearance. Like their counterparts in the circulatory system, they range in size from lymphatic capillaries to vessels of increasing diameter. Like veins, lymphatics have intermittent valves composed of folds of their inner layer that extend into the lumen (Figure 15-7). The walls of lymphatic capillaries contain contractile fibers that are stimulated when stretched, causing the vessels to contract and propel lymph along the vessel.
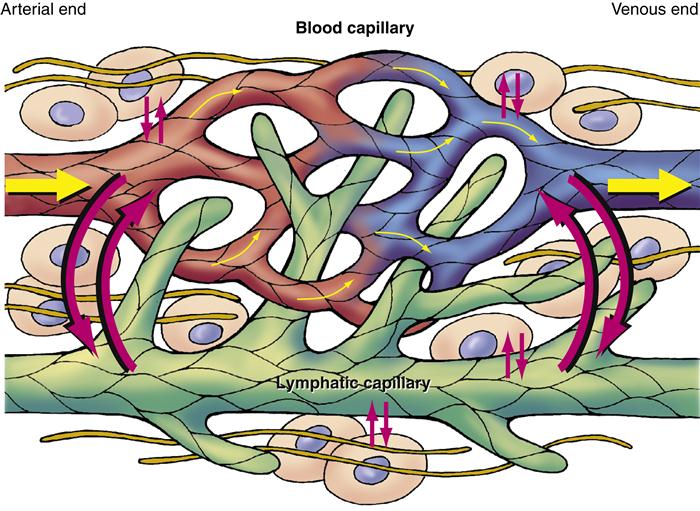
The lymphatic system is integrally related to the systemic vascular system. Excess fluid and plasma diffuse between the capillaries, interstitial spaces, and lymphatic vessels. Because lymphatic capillaries have larger spaces between endothelial cells, they can remove excess interstitial fluid or plasma that venous capillaries cannot reabsorb.
Principles of Flow
Hemodynamics of the Circulatory System
The principles of blood flow are known as circulatory hemodynamics. These principles govern the quantity of blood passing by a given point in a specific period. Therefore, blood flow is measured as a given number of liters, milliliters, or cubic centimeters per second, minute, or hour. A discussion of the hemodynamics of the circulatory system includes the concepts of pressure, resistance, velocity, laminar and turbulent flows, wall tension, and compliance.
Blood Flow, Pressure, and Resistance
Blood flow is accomplished by movement along a pressure gradient within the vascular bed. This means that blood moves from an area of higher pressure to an area of lower pressure. The arterial and arteriolar walls with their muscular media coats provide the high-pressure end of the gradient. Seeking a lower pressure, blood moves toward the venous system. The thinner, more pliable walls of the venous bed furnish the low-pressure portion of the pressure gradient. The greater the pressure difference, the greater the rate of blood flow.
The movement of blood through the vascular system is opposed by the force of resistance. The relationship between blood flow and resistance is an inverse one: as resistance increases, blood flow decreases. This force has several determinants, each of which can change resistance considerably; these determinants are represented in physiology by Poiseuille’s law:

The number 8 is a mathematical constant, as is the value of π; n represents blood viscosity, l represents the length of a given vessel, and r is the radius of the vessel. Using this formula, the effects of changes on the components of resistance are very predictable.
Two of the determinants of resistance are vessel length and vessel radius. As predicted by Poiseuille’s law, resistance changes directly with the length of the vessel, and these changes in resistance significantly affect flow. As illustrated in Figure 15-8, given three vessels of the same radius, doubling the length increases the resistance and reduces the flow (Q) by 50%. Reducing the vessel length by half decreases resistance and increases the flow by 100%. These changes in flow occur when the pressure gradient remains constant and are caused solely by variations in vessel length. Resistance decreases as the radius of a vessel increases and resistance is inversely related to the fourth power of the radius of a vessel, or r4. Therefore, increasing a vessel’s radius markedly reduces resistance and produces an exponential increase in blood flow. Figure 15-9 demonstrates the effect of doubling the radius of a vessel on the flow of blood if all other factors related to flow are held constant. The resulting flow of blood is 16 times greater in the greater diameter vessel.
Although there is variability in the length of vessels throughout the circulatory system, vessels are incapable of altering their own length. They do, however, possess a considerable ability to change their diameters, and many disease processes (e.g., arteriosclerosis) and drug therapies (e.g., vasopressors) are associated with changes in the size of the vessel lumen. Even minor changes will produce major alterations in resistance and, hence, blood flow. This makes changes in diameter the most important determinant of resistance.
The third determinant of resistance is the viscosity of the blood itself, represented in Poiseuille’s law as n. Viscosity is defined as the thickness of a fluid. When the blood is more viscous, the friction between the cells and the liquid increases, and an increase in resistance to flow is produced. Blood is composed of a suspension of cellular material and plasma. Approximately 99% of the cellular constituents of the blood are RBCs. The ratio of RBCs to plasma is presented in the laboratory value hematocrit. Increasing the number of RBCs or decreasing the plasma component results in more viscous blood (increased hematocrit value), more resistance, and a slowing of blood flow. This is what occurs in dehydration, when the plasma component is relatively decreased, or in polycythemia, when the number of RBCs increases.
The relationship between the variables of driving pressure and resistance and their effect on blood flow is expressed by Ohm’s law, as follows:

Here, Q is the blood flow, P is the pressure difference between two points, and R is resistance. Altering any one of the determinants of resistance (vessel length, vessel radius, or blood viscosity) produces a change in flow. According to Ohm’s law, a change in the pressure difference within the circulatory system also results in a change in the flow of blood. The arterioles are the major site of resistance in the vascular system and require a greater pressure to maintain blood flow. As the resistance decreases across the systemic vasculature, less pressure is necessary to maintain blood flow (Figure 15-10). Total peripheral resistance refers to the resistance throughout the entire vascular system. It can be calculated on the basis of the pressure difference between the arteries and the veins. Clinically, systemic vascular resistance (SVR) is used to specifically denote resistance peripheral to the heart and lungs. Because the primary determinant of SVR is the resistance vessels (arterioles), diseases or drug therapies that affect these vessels have the most profound impact on the SVR. Any condition that produces an increase in SVR, such as hypertension, requires more work for the heart to overcome the elevated resistance and eject its volume of blood (see Chapter 16). This increased workload means that the heart needs more oxygen and nutrients. When SVR is pathologically decreased, the blood is distributed over a larger area and blood flow slows dramatically. Individual organs, such as the kidney and brain, may not obtain sufficient blood flow to meet metabolic needs. This is what occurs in distributive shock states (see Chapter 20).
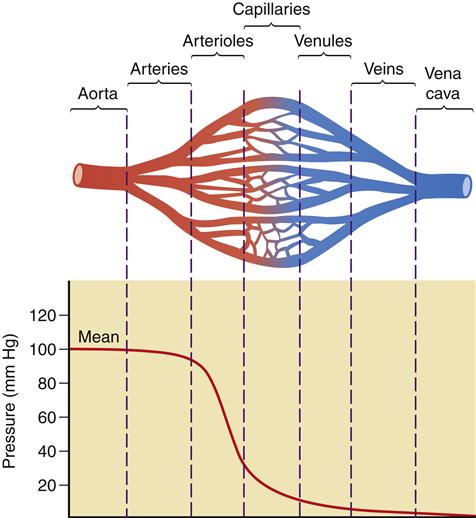
A significant decrease in pressure occurs as blood flows through the arterioles into the capillaries. The figure illustrates the role of the arterioles in the determination of vascular resistance. Because of the large number of capillaries, total resistance is not increased with the decreased radius of the capillaries.
Velocity and Laminar and Turbulent Flow
As previously discussed, blood flow is defined as the volume of blood that passes by a given point in a given unit of time. Velocity is a measure of the distance traveled in a given interval of time and is usually expressed in centimeters per second. Velocity is governed by the total cross-sectional area and varies inversely with it. An increase in the total cross-sectional area produces a decrease in velocity, whereas a decrease in the total cross-sectional area produces an increase in velocity. The total cross-sectional area of the aorta and vena cava is small and they have the most rapid rate of flow, whereas the capillary beds combine to produce the greatest total cross-sectional area and have the slowest flow rate. The dividing and subdividing of vessels within the circulatory system results in greater velocity in the arterial and venous beds than in the capillary bed (Figure 15-11). An understanding of the concept of velocity enhances discussion of laminar and turbulent flow.
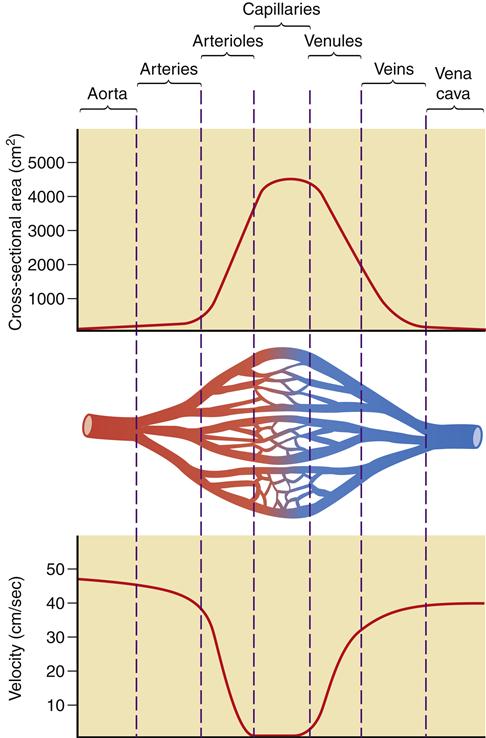
Increased cross-sectional area in the capillary bed results in a significant decrease in velocity when compared to the arterial and venous networks.
When blood flows through a long, smooth-walled vessel, it does so in layers. The velocity of the layers varies, with blood in the center moving much faster than blood in the outer layers. The blood in the center layer moves the most quickly because it is in contact with blood only. The outermost layer is also in contact with the intima of the vessel wall, which exerts friction against the cellular components of the blood. Many blood cells stick to the intima; this layer may flow only minimally. Layers of blood between this outer layer and the central core of blood slide over one another with increasing velocity. This is referred to as the parabolic profile of laminar flow and is illustrated in Figure 15-12.
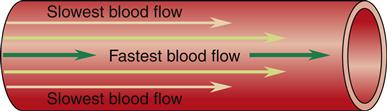
The streamlined nature of laminar flow is disrupted by normal anatomy and by pathologic processes creating turbulent flow. Turbulent flow is an interruption in the forward current of blood flow by crosswise flow (Figure 15-13). The propensity for turbulent flow increases with increasing velocity and increased vessel radius, so that some turbulence can be predicted at the aortic root and in the branches of major arteries. The same process can be seen in a river, where boulders interrupting the flow produce whirlpools and the characteristic roar of rapids. In the human body, turbulent flow through blood vessels can be auscultated as a bruit. Sometimes it can be palpated as well, and then it is called a thrill. This turbulence may be the result of a normal increase in velocity or be attributable to blood moving through vessels that branch at a sharp angle. Pathologically, turbulence results if blood flows around an obstruction in the vessel or over a roughened intimal surface. Regardless of cause, turbulent flow alters the parabolic profile seen with laminar flow, slowing velocity around the source of the turbulence. This slowing can cause cellular components of the blood to adhere to one another, to the turbulent focus itself, and to the intimal wall, promoting the formation of a blood clot (thrombus).
Wall Tension and Compliance
The relationship between distending pressure and wall tension is expressed by the law of Laplace and is illustrated in Figure 15-14. This physical principle has broad applications in physiology; however, the present discussion focuses on its implications for blood vessels. The distending pressure (P) is the transmural pressure, or pressure on one side of the vessel wall minus the pressure on the other side of the blood vessel. It is equal to the wall tension (T) divided by the radius of the blood vessel (r). In summary, an increase in radius or distending pressure results in increased wall tension.
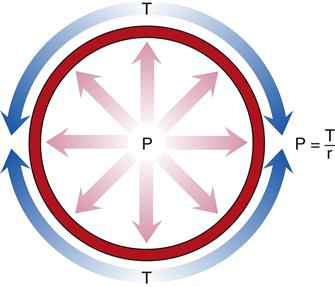
Distending pressure (P) is the difference between the pressures on either side of the vessel and is equal to the wall tension (T) divided by the radius of the blood vessel (r).
When the pressure of the blood in the vessel begins to decline, wall tension forces exceed distending forces, the radius decreases, flow rate declines, and resistance increases. The distending pressure may fall to a point at which it is no longer possible to hold the blood vessel open. If the pressure reaches 20 mm Hg, a point called the critical closing pressure, blood flow ceases entirely.
The smaller the radius of the blood vessel, as in a capillary when compared to an artery or a vein, the less tension is needed in the wall to equalize the distending pressure. Wall tensions decrease rapidly from 170,000 dynes/cm in the aorta to 16 dynes/cm in the capillaries, rising to 21,000 dynes/cm in the vena cava.
Wall tension is a product of the elasticity of the vessel and is a force that opposes the distending pressure. The manner in which wall tension in a given vessel responds to changes in distending pressures is based on its compliance. Compliance reflects the distensibility of a blood vessel—its ability to accept an increased volume of blood. The large quantity of muscle tissue in much of the arterial system limits its distensibility. Veins, however, are highly distensible and compliant, capable of holding a large quantity of blood at a low pressure. Because of this quality, veins are referred to as capacitance vessels. When the body is at rest, 75% of the total blood volume is found in the systemic venous system.
Dynamics in the Microcirculation: Capillaries and Lymphatics
The smallest vessels of the vascular system and the lymphatic vessels are commonly referred to as the microcirculation. The primary function of the capillary bed is essentially the essence of the entire circulatory system: the exchange of gases and nutrients. Blood flow in the capillary bed is largely laminar, with minimal turbulence at bifurcations. Within each organ or tissue in the body, capillary blood flow is related to the driving force, which is the difference between arterial and venous pressures, and inversely related to resistance.
The exchange of materials across the capillary endothelium through the interstitial space, to or from the cells, occurs on an ongoing basis. Substances pass between tissue interstitial fluid and capillary blood by moving along a concentration gradient (diffusion), whereas fluid moves according to a pressure gradient (filtration). As fluid moves through the interstitial space, most of it returns to the capillary bed. Normally, approximately 10% of the fluid remains in the interstitium and is absorbed by the adjacent lymphatic system to be returned to the general circulation. Alteration in the pressure gradient responsible for filtration can allow an excessive amount of fluid to escape into the interstitial space. Increased fluid accumulation in the interstitial space also can occur when the lymphatic flow is impaired or when capillaries become more permeable and “leak” fluid. These are the pathophysiologic mechanisms that result in edema.
The pressure gradient between the capillary and the interstitium is produced and maintained in accord with the balance of four distinct forces or pressures: (1) capillary hydrostatic pressure (Pcap), (2) interstitial fluid colloid osmotic pressure (πtissue), (3) plasma colloid osmotic pressure (πcap), and (4) interstitial fluid pressure (Ptissue) (Figure 15-15). This delicate balance of forces is summarized by Starling’s hypothesis, which states that the net filtration is equal to the combined forces fostering filtration minus the combined forces opposing filtration. Using the normal values shown in Figure 15-15, each component of the formula and the resulting net filtration pressure are shown below:






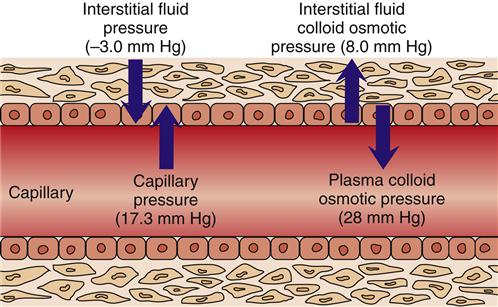
Filtration reflects the difference between the combined forces that push fluid out of the capillary (capillary pressure and interstitial fluid colloid osmotic pressure) and those that attempt to hold fluid in the capillary (plasma colloid osmotic pressure and interstitial fluid pressure).
Clinically, capillary fluid pressure and plasma colloid osmotic pressure are the most important concepts to a discussion of pathophysiology. Capillary fluid pressure is the blood pressure in the capillary. It is the force pushing fluid from the capillary into the interstitium and is often called the hydrostatic pressure. The strength of this force depends on the blood pressure and the resistance within the arterial and venous systems. Pathologic conditions resulting in an increase in either the blood pressure or the resistance to flow can alter this force, increasing it and propelling more fluid into the interstitial space, resulting in the formation of edema.
Plasma proteins are responsible for the plasma colloid osmotic pressure, the primary force resulting in fluid remaining in the capillary. Most plasma proteins normally remain in the capillaries because they are such large molecules that they cannot move through the capillary spaces. The vast majority of plasma protein, by weight, is albumin. Although globulins and fibrinogen have greater molecular weight, albumin is present in plasma in greater quantity. The number of dissolved molecules in the plasma determines the plasma colloid osmotic pressure. The number of dissolved molecules in the interstitial space establishes the interstitial fluid colloid osmotic pressure. Plasma has nearly four times the concentration of proteins than does the interstitium. For that reason, plasma colloid osmotic pressure normally exceeds that in the interstitium, favoring fluids remaining in the capillaries.
As previously illustrated, the net filtration pressure in a typical capillary is 0.3 mm Hg. This pressure difference is responsible for producing the fluid excess in the interstitial space, which is then normally absorbed by the lymphatic system for eventual return to the systemic vascular circulation. If the pressures are altered, an even greater pressure gradient may be produced and more fluid moves from the capillaries into the interstitial space. Likewise, a change in the permeability (K) of the capillary wall that allows plasma proteins to leak out, or a reduction in lymphatic flow will allow fluid to collect in the interstitium. In each case, the result is edema, which can occur with many pathologic conditions. When the pathology is an impairment of lymphatic flow allowing fluid to collect in the interstitium, it is more specifically termed lymphedema.
Once absorbed into the lymphatic system, interstitial fluid is referred to as lymph. It is similar in composition to interstitial fluid but has a lower concentration of protein. Molecules of fat and bacteria are also found in lymph. Lymph circulates throughout the body at a rate of approximately 3 L/day. Lymphatic flow can be increased in several ways: by increasing the capillary pressure, decreasing the plasma colloid osmotic pressure, increasing the interstitial fluid colloid osmotic pressure, or increasing the permeability of the capillaries. The interstitial fluid hydrostatic pressure increases (becomes less negative) when any of these factors changes, producing an increase in lymphatic flow.1
Control of Flow
Blood flow throughout the periphery is controlled by central mechanisms that are mediated by the autonomic nervous system, the venous and thoracic pumps, and intrinsic autoregulatory mechanisms. Lymphatic flow is controlled by increasing interstitial fluid colloid osmotic pressure and by the stimulation of the contractile fibers (often called lymphatic pumps) as they are stretched. In healthy people, these mechanisms of control respond to changes in the internal and external environments and compensate rapidly and efficiently; however, during states of illness these mechanisms may be inadequate to compensate for alterations in flow.
Control of Blood Flow
Extrinsic Mechanisms
The autonomic nervous system provides the primary extrinsic control of blood flow through the sympathetic nervous system (SNS). Although parasympathetic nervous system (PSNS) innervation is important to the regulation of the heart, it is not important to the regulation of peripheral resistance. Within the medulla, groups of neurons form the vasomotor center. This area plays a major role in the maintenance of blood pressure (see Chapter 16). The vasomotor center responds to direct stimulation and to afferent stimuli of both an excitatory and an inhibitory nature. A basal rate of discharge from the vasomotor center results in a continuous minimal level of contraction of vascular smooth muscle, referred to as vasomotor tone.
All blood vessels except the small venules and capillaries contain smooth muscle that is innervated by adrenergic fibers from the SNS. Because arteries have the most smooth muscle, they are most affected by SNS stimulation. Veins, by contrast, have little neural innervation, and venoconstriction has a minor role in controlling blood flow except in the skin and the splanchnic circulatory bed of the gut. In general, the release of norepinephrine, the SNS postganglionic neurotransmitter, results in arterial vasoconstriction via the α1 receptors located on the vascular smooth muscle walls. Likewise, drugs that mimic the α1-receptor response (α1 agonists) produce vasoconstriction, increasing vasomotor tone and diastolic blood pressure. Administration of an α1 antagonist results in the blockade of these receptors and results in vasodilation of the arterial bed, reducing blood pressure.
Although the β2-adrenergic receptors located on blood vessels in skeletal muscle produce vasodilation when stimulated, they are only minimally affected by endogenous norepinephrine from the SNS. Epinephrine, the endogenous catecholamine released by the adrenal medulla, or its exogenous pharmacologic equivalent (adrenalin), stimulates these receptors, producing vasodilation. Therefore, their major role is not so much to maintain vasomotor tone but to increase nutrient and oxygen supplies to skeletal muscles during periods of increased demand.
Blood flow through the venous system into the right side of the heart is maintained by the pressure gradient from the veins and by the venous and thoracic pumps. Blood is propelled through the circuit, pushed by the force of left ventricular contraction, and moves forward toward the low-pressure side of the pump on the right side of the heart. In the peripheral veins, what is known as the venous pump is activated by skeletal muscle activity. Folds in the intimal wall of the veins create valves. Contraction of the skeletal muscles bordering the veins compresses them, forcing the valves open and propelling venous blood back toward the heart. Instigation of the venous pump significantly facilitates venous return. Patients who are immobilized by bed rest lose this valuable mechanism, which results in a decrease in cardiac preload to the right heart and increased work of the heart to maintain the cardiac output. The thoracic pump acts to increase venous return to the heart as intrathoracic pressure changes with breathing. Inspiration increases negative intrathoracic pressure, resulting in more venous return (see Chapter 17).
Intrinsic Mechanisms
Autoregulation refers to the ability of blood vessels within organs to maintain a relatively constant blood flow, regardless of changes in arterial pressure. This flow is relatively constant because it does have limits; there is a range within which it is maintained, and the range varies slightly from organ to organ. Several processes contribute to the autoregulation of perfusion to meet the needs of individual organs within the body.
It is known that as vascular smooth muscle is stretched, it contracts. Therefore, as arterial pressure rises and arterial walls stretch, contraction is stimulated, producing vasoconstriction. Resistance to flow is also increased with stretch by early closing of precapillary sphincters. This process certainly may contribute to autoregulation, but it is not the primary mechanism.
Over the past several decades, a great deal has been learned about the endothelium of blood vessels. The previous perceptions of the endothelium as an inactive structure, whose function was no more than acting as a barrier between the blood and the more functional layers of the vessel wall, have been proven incorrect.2 We now understand that the endothelium is a major participant in vascular tone and growth of vascular smooth muscle.2 The endothelium tissue plays an active role in the immune and inflammatory processes (see Chapter 9), platelet activity in normal coagulation and thrombus formation (see Chapter 14), and arteriosclerosis,2–4 discussed later in this chapter. In a discussion of autoregulation, the key is the endothelial role in modulating vascular smooth muscle to produce vasoconstriction or vasodilation. The endothelium is capable of sensing alterations of a chemical or physical nature within the vessel and responding to these stimuli directly or through the release of signals that initiate change.3 Almost all of the vasodilation occurs because of the effect of nitric oxide (NO). NO is a gas present in most body tissues and is produced by the cells of the vascular endothelium. As a gas, it diffuses from the endothelium to the smooth muscle cells, binding to intracellular receptors to impact cytoplasmic Ca++ concentration and produce vasodilation.3–5 Deficits of nitric oxide, or a decreased responsiveness, have been the focus of considerable recent research into the pathogenesis of hypertension (Chapter 16) and heart disease (Chapter 18).2–7 Other relaxing factors produced by the endothelium include prostacyclin, and endothelium-derived hyperpolarizing factor.6 Angiotensin II, endothelin, oxygen-derived free radicals, prostacyclin H2, and thromboxane A2 are among the constricting factors. The role of angiotensin II (AII) as a constricting factor has been the focus of considerable recent research.7 Because drugs that block the effects of AII are available and in widespread use, the ability of these drugs to improve peripheral vascular blood flow has been an important finding.7 In addition to substances produced by the endothelium itself, metabolic by-products (metabolites) or substrates have been found to exert a direct effect, altering blood flow to the area.1 Metabolites might include carbon dioxide or lactic acid. Histamine and prostaglandins are examples of metabolic substrates. Other substances, such as acetylcholine, bradykinin, histamine, and substance P, exert their effect by increasing the formation of nitric oxide or are themselves generated by nitric oxide.7 These various chemicals create a balance of forces in health. They may also be affected by aging, disease, or pharmacologic interventions.
A local increase in blood flow is referred to as hyperemia. The increase in local blood flow in response to increased metabolic demand is called active or functional hyperemia. Reactive hyperemia occurs when a temporary reduction in blood flow is reversed. The body responds by briefly increasing circulation to the area, resulting in the characteristic flushing seen, for instance, when a tourniquet is removed. The tissue pressure hypothesis of autoregulation postulates that an acute increase in the pressure within the arterial system causes an increase in interstitial volume and pressure. This increased tissue pressure, external to the vasculature, results in compression of small vessels, which increases resistance and reduces flow.
Control of Lymphatic Flow
The movement of lymph is expedited by lymphatic pumps. This is a general concept that encompasses the pumping action of the lymphatics themselves and the pumping effect on the lymphatic vessels produced by activity external to them. Like veins, lymphatic vessels have valves on their intimal surface that allow forward movement of fluid to join the venous return to the systemic circulation. Compression of lymphatic channels by adjacent skeletal muscles, the smooth muscle of organs, and the pulsatile movement of arteries force lymph forward. Intrathoracic pressure changes related to breathing increase lymphatic return as well as venous return. Lymphatic flow is therefore enhanced by increased physical activity, increased blood pressure, or increased respiratory rate. Lymphatic contractions are thought to be the primary factor in lymphatic flow. Lymph is propelled forward when lymphatic capillaries contract in response to being stretched. The rate of contractions increases as the volume of lymph increases.