Many hormones affect fuel metabolism, including those that regulate appetite as well as those that influence absorption, transport, and oxidation of foodstuffs. The major hormones that influence nutrient metabolism and their actions on muscle, liver, and adipose tissue are listed in Table 41.1.
TABLE 41.1 Major Hormones That Regulate Fuel Metabolism
↑↑, pronounced increased effect; ↑, moderate increased effect; ↓, moderate decreased effect; –, no effect.
aHormones with actions that are generally opposed to those of insulin.
bPermissive: allowing and enhancing the response by other hormones.
cSomatostatin’s effects on metabolism are indirect via suppression of secretion of insulin, glucagon, growth hormone, and thyroid hormone and by effects on gastric acid secretion, gastric emptying time, and pancreatic exocrine secretion (see text).
Insulin is the major anabolic hormone. It promotes the storage of nutrients as glycogen in liver and muscle, and as triacylglycerols in adipose tissue. It also stimulates the synthesis of proteins in tissues such as muscle. At the same time, insulin acts to inhibit fuel mobilization.
Glucagon is the major counterregulatory hormone. The term “counterregulatory” means that its actions are generally opposed to those of insulin (contrainsular). The major action of glucagon is to mobilize fuel reserves by stimulating glycogenolysis and gluconeogenesis. These actions ensure that glucose will be available to glucose-dependent tissues between meals.
Epinephrine, norepinephrine, cortisol, somatostatin, and growth hormone also have contrainsular activity. Thyroid hormone also must be classified as an insulin counterregulatory hormone because it increases the rate of fuel consumption and also increases the sensitivity of the target cells to other insulin counterregulatory hormones.
Insulin and the counterregulatory hormones exert two types of metabolic regulation (see Chapter 19). The first type of control occurs within minutes to hours of the hormone–receptor interaction and usually results from changes in the catalytic activity or kinetics of key pre-existing enzymes, caused by phosphorylation or dephosphorylation of these enzymes. The second type of control involves regulation of the synthesis of key enzymes by mechanisms that stimulate or inhibit transcription and translation of messenger RNA (mRNA). These processes are slow and require hours to days.
THE WAITING ROOM 
Otto S., now a third-year medical student, was assigned to do a history and physical examination on a newly admitted 47-year-old patient named Chet S. had consulted his physician for increasing weakness and fatigue and was found to have a severely elevated serum glucose level. While he was examining the patient, Otto S. noted marked redness of Chet S.’s facial skin as well as reddish-purple stripes (striae) in the skin of his lower abdomen and thighs. The patient’s body fat was unusually distributed in that it appeared to be excessively deposited centrally in his face, neck, upper back, chest, and abdomen, while the distal portions of his arms and legs appeared to be almost devoid of fat. Chet S.’s skin appeared thinned and large bruises were present over many areas of his body, for which Chet S. had no explanation. The neurological examination showed severe muscle weakness, especially in the proximal arms and legs, where the muscles seemed to have atrophied.
Sam A., a 42-year-old jeweler, noted increasingly severe headaches behind his eyes that were sometimes associated with a “flash of light” in his visual field. At times, his vision seemed blurred, making it difficult to perform some of the intricate work required of a jeweler. He consulted his ophthalmologist, who was impressed with the striking change in Sam A.’s facial features that had occurred since he last saw the patient 5 years earlier. The normal skin creases in Sam A.’s face had grown much deeper, his skin appeared to be thickened, his nose and lips appeared more bulbous, and his jaw seemed more prominent. The doctor also noted that Sam A.’s hands appeared bulky, and his voice had deepened. An eye examination showed that Sam A.’s optic nerves appeared slightly atrophied, and there was a loss of the upper outer quadrants of his visual fields.
I. Physiologic Effects of Insulin and Amylin
The effects of insulin on fuel metabolism and substrate flux were considered in many of the earlier chapters of this book, particularly in Chapter 19. Insulin stimulates the storage of glycogen in liver and muscle and the synthesis of fatty acids and triacylglycerols and their storage in adipose tissue. In addition, insulin stimulates the synthesis in various tissues of >50 proteins, some of which contribute to the growth of the organism. In fact, it is difficult to separate the effects of insulin on cell growth from those of a family of proteins known as the somatomedins or the insulin-like growth factors I and II (IGF-I and IGF-II) (see Section III.B.6).
Finally, insulin has paracrine actions within the pancreatic islet cells. When insulin is released from the β-cells, it suppresses glucagon release from the α-cells.
Amylin is a 37 amino acid peptide, which is synthesized in the β-cells of the pancreas and is cosecreted with insulin when blood glucose levels rise. Amylin has been demonstrated to suppress the postprandial glucagon secretion (thereby enhancing the effect of insulin, by keeping the insulin:glucagon ratio high), to slow gastric emptying, which decreases the rate at which food reaches the intestine, and the bloodstream (thereby blunting a large increase in blood nutrient levels immediately after a meal), and to reduce food intake (and thereby body weight). All of amylin’s actions are geared toward reducing blood glucose levels, just as insulin does when it is released from the pancreas. Individuals with type 1 diabetes, who experience destruction of the β-cells of the pancreas, lose the ability to secrete both insulin and amylin in response to an increase in blood nutrient levels, and may partially explain why insulin therapy for individuals with type 1 diabetes is often inadequate in preventing the long-term complications of diabetes.
II. Physiologic Effects of Glucagon
Glucagon is one of several counterregulatory (contrainsular) hormones. It is synthesized as part of a large precursor protein called proglucagon. Proglucagon is produced in the α-cells of the islets of Langerhans in the pancreas and in the L-cells of the intestine. It contains a number of peptides linked in tandem: glicentin-related peptide, glucagon, glucagon-related peptide 1 (GLP-1), and glucagon-related peptide 2 (GLP-2). Proteolytic cleavage of proglucagon releases various combinations of its constituent peptides. Glucagon is cleaved from proglucagon in the pancreas and constitutes 30% to 40% of the immunoreactive glucagon in the blood. The remaining immunoreactivity is caused by other cleavage products of proglucagon released from the pancreas and the intestine. Pancreatic glucagon has a plasma half-life of 3 to 6 minutes and is removed mainly by the liver and kidney.
Glucagon promotes glycogenolysis, gluconeogenesis, and ketogenesis by stimulating the generation of cyclic adenosine monophosphate (cAMP) in target cells. The liver is the major target organ for glucagon, in part because the concentrations of this hormone bathing the liver cells in the portal blood are higher than in the peripheral circulation. Levels of glucagon in the portal vein may reach concentrations as high as 500 pg/mL.
Finally, glucagon stimulates insulin release from the β-cells of the pancreas. Whether this is a paracrine effect or an endocrine effect has not been established. The pattern of blood flow in the pancreatic islet cells is believed to bathe the β-cells first and then the α-cells. Therefore, the β-cells may influence α-cell function by a paracrine mechanism, whereas the influence of α-cell hormone on β-cell function is more likely to be endocrine. The glucagon-stimulated insulin release is most likely necessary to maintain blood glucose levels in the physiologic range.
III. Physiologic Effects of Other Counterregulatory Hormones
A. Somatostatin
1. Biochemistry
Preprosomatostatin, a 116-amino acid peptide, is encoded by a gene located on the long arm of chromosome 3. Somatostatin (SS-14), a cyclic peptide with a molecular weight of 1,600 Da, is produced from the 14 amino acids at the C-terminus of this precursor molecule. SS-14 was first isolated from the hypothalamus and named for its ability to inhibit the release of growth hormone (GH, somatotropin) from the anterior pituitary. It also inhibits the release of insulin. In addition to the hypothalamus, somatostatin is also secreted from the D cells (δ-cells) of the pancreatic islets, many areas of the central nervous system outside of the hypothalamus, and gastric and duodenal mucosal cells. SS-14 predominates in the central nervous system (CNS) and is the sole form secreted by the δ-cells of the pancreas. In the gut, however, a longer form of somatostatin is produced (SS-28), which has 14 additional amino acids extending from the C-terminal portion of the precursor, and makes up 70% to 75% of the immunoreactivity (the amount of hormone that reacts with antibodies to SS-14). The prohormone SS-28 is 7 to 10 times more potent in inhibiting the release of GH and insulin than is SS-14.
2. Secretion of Somatostatin
The secretagogues for somatostatin are similar to those that cause secretion of insulin. The metabolites that increase somatostatin release include glucose, arginine, and leucine. The hormones that stimulate somatostatin secretion include glucagon, vasoactive intestinal polypeptide (VIP), and cholecystokinin (CCK). Insulin, however, does not influence somatostatin secretion directly.
3. Physiologic Effects of Somatostatin
Somatostatin and its synthetic analogs are used clinically to treat a variety of secretory neoplasms such as GH-secreting tumors of the pituitary. Such tumors can cause gigantism if GH is secreted in excess before the closure of the growth centers of the ends of long bones or acromegaly if excess GH is chronically secreted after the closure of these centers (as in Sam A.).
B. Growth Hormone
1. Biochemistry
Growth hormone (GH) is a polypeptide that, as its name implies, stimulates growth. Many of its effects are mediated by insulin-like growth factors (IGFs, also known as somatomedins) that are produced by cells in response to the binding of GH to its cell membrane receptors (see Section III.B.6). However, GH also has direct effects on fuel metabolism.
Human GH is a water-soluble 22-kDa polypeptide with a plasma half-life of 20 to 50 minutes. It is composed of a single chain of 191 amino acids having two intramolecular disulfide bonds (Fig. 41.1). The gene for GH is located on chromosome 17. It is secreted by the somatotroph cells (the cells that synthesize and release GH) in the lateral areas of the anterior pituitary. GH is structurally related to human prolactin and to human chorionic somatomammotropin (hCS) from the placenta, a polypeptide that stimulates growth of the developing fetus. Yet the hCS peptide has only 0.1% of the growth-inducing potency of GH. GH is the most abundant trophic hormone in the anterior pituitary, being present in concentrations of 5 to 15 mg/g of tissue. The other anterior pituitary hormones are present in quantities of micrograms per gram of tissue.
FIGURE 41.1 Structure of human growth hormone. (Republished with permission of McGraw Hill LLC from Murray RK, Granner DK, Mayes PA, et al. Harper’s Biochemistry. 23rd ed. Appleton & Lange; 1993:502; permission conveyed through Copyright Clearance Center, Inc.)
The IGF-I–independent actions of GH are exerted primarily in hepatocytes. GH administration is followed by an early increase in the synthesis of 8 to 10 proteins, among which are IGF-I, α2-macroglobulin, and the serine protease inhibitors Spi 2.1 and Spi 2.3. Expression of the gene for ornithine decarboxylase, an enzyme that is active in polyamine synthesis (and, therefore, in the regulation of cell proliferation), is also significantly increased by GH.
Muscle and adipocyte cell membranes contain GH receptors that mediate direct, rapid metabolic effects on glucose and amino acid transport as well as on lipolysis. These receptors use associated cytoplasmic tyrosine kinases for signal transduction (such as the Janus kinases; see Chapter 10, Section XII.B). STAT (signal transducer and activator of transcription) transcription factors are activated, and, depending on the tissue, the MAP kinase pathway, or the AKT pathway is also activated. For example, in adipose tissue, GH has acute insulin-like effects followed by increased lipolysis, inhibition of lipoprotein lipase, stimulation of hormone-sensitive lipase, decreased glucose transport, and decreased lipogenesis. In muscle, GH causes increased amino acid transport, increased nitrogen retention, increased fat-free (lean) tissue, and increased energy expenditure. GH also has growth-promoting effects. GH receptors are present on a variety of tissues in which GH increases IGF-I gene expression. The subsequent rise in IGF-I levels contributes to cell multiplication and differentiation by autocrine or paracrine mechanisms. These, in turn, lead to skeletal, muscular, and visceral growth. These actions are accompanied by a direct anabolic influence of GH on protein metabolism with a diversion of amino acids from oxidation to protein synthesis and a shift to a positive nitrogen balance.
2. Control of Secretion of Growth Hormone
Although the regulation of GH secretion is complex, the major influence is hormonal (Fig. 41.2). The pulsatile pattern of GH secretion reflects the interplay of two hypothalamic regulatory peptides. Release is stimulated by growth hormone–releasing hormone (GHRH, also called somatocrinin). The structure of GHRH was identified in 1982 (Fig. 41.3). It exists as both a 40- and a 44-amino acid peptide encoded on chromosome 20 and produced exclusively in cells of the arcuate nucleus. Its C-terminal leucine residue is amidated. Full biological activity of this releasing hormone resides in the first 29 amino acids of the N-terminal portion of the molecule. GHRH interacts with specific receptors on the plasma membranes of the somatotrophs. The intracellular signaling mechanisms that result in GH synthesis and release appear to be multiple, as cAMP and calcium–calmodulin both stimulate GH release.
FIGURE 41.2 Control of growth hormone secretion. Various factors stimulate the release of growth hormone–releasing hormone (GHRH) from the hypothalamus. The hypothalamus also releases somatostatin in response to other stimuli. GHRH stimulates and somatostatin inhibits the release of growth hormone from the somatotrophs of the anterior pituitary. Growth hormone causes the release of insulin-like growth factor-1 (IGF-I) from liver and other tissues. IGF-I inhibits GHRH release and stimulates somatostatin release.
FIGURE 41.3 Structures of growth hormone–releasing hormone (GHRH) and growth hormone release–inhibiting hormone (GHRIH, the same as somatostatin). GHRH has an amide at the C-terminal (in box).
Conversely, GH secretion is suppressed by growth hormone release–inhibiting hormone (GHRIH, the same as somatostatin, which has already been discussed). In addition, IGF-I, produced primarily in the liver in response to the action of GH on hepatocytes, feeds back negatively on the somatotrophs to limit GH secretion. Other physiologic factors (e.g., exercise and sleep) and many pathologic factors also control its release (Table e-41.1) .
In addition, GH release is modulated by plasma levels of all of the metabolic fuels, including proteins, fats, and carbohydrates. A rising level of glucose in the blood normally suppresses GH release, whereas hypoglycemia increases GH secretion in normal subjects. Amino acids, such as arginine, stimulate release of GH when their concentrations rise in the blood. Rising levels of fatty acids may blunt the GH response to arginine or a rapidly dropping blood glucose level. However, prolonged fasting, in which fatty acids are mobilized in an effort to spare protein, is associated with a rise in GH secretion. Some of the physiologic, pharmacologic, and pathologic influences on GH secretion are given in Table e-41.1 . These modulators of GH secretion provide the basis for clinical suppression and stimulation tests in patients suspected of having excessive or deficient GH secretion.
3. Effects of Growth Hormone on Energy Metabolism
GH affects the uptake and oxidation of fuels in adipose tissue, muscle, and liver and indirectly influences energy metabolism through its actions on the islet cells of the pancreas. In summary, GH increases the availability of fatty acids, which are oxidized for energy. This and other effects of GH spare glucose and protein; that is, GH indirectly decreases the oxidation of glucose and amino acids (Fig. 41.4).
FIGURE 41.4 Anabolic effects of growth hormone on various tissues. GHRH, growth hormone–releasing hormone; IGF-I, insulin-like growth factor-1.
4. Effects of Growth Hormone on Adipose Tissue
GH increases the sensitivity of the adipocyte to the lipolytic action of the catecholamines and decreases its sensitivity to the lipogenic action of insulin. These actions lead to the release of free fatty acids and glycerol into the blood to be metabolized by the liver. GH also decreases esterification of fatty acids, thereby reducing triacylglycerol synthesis within the fat cell. Recent evidence suggests that GH may impair glucose uptake by both fat and muscle cells by a postreceptor inhibition of insulin action. As a result of the metabolic effects of GH, the clinical course of acromegaly (increased GH secretion) may be complicated by impaired glucose tolerance or even overt diabetes mellitus.
5. Effects of Growth Hormone on Muscle
The lipolytic effects of GH increase free fatty acid levels in the blood that bathes muscle. These fatty acids are used preferentially as fuel, indirectly suppressing glucose uptake by muscle cells. Through the effects on glucose uptake, the rate of glycolysis is proportionately reduced.
GH increases the transport of amino acids into muscle cells, providing substrate for protein synthesis. Through a separate mechanism, GH increases the synthesis of DNA and RNA. The positive effect on nitrogen balance is reinforced by the protein-sparing effect of GH-induced lipolysis that makes fatty acids available to muscle as an alternative fuel source.
6. Effects of Growth Hormone on the Liver
When plasma insulin levels are low, as in the fasting state, GH enhances fatty acid oxidation to acetyl CoA. This effect, in concert with the increased flow of fatty acids from adipose tissue, enhances ketogenesis. The increased amount of glycerol reaching the liver as a consequence of enhanced lipolysis acts as a substrate for gluconeogenesis.
Hepatic glycogen synthesis is also stimulated by GH in part because of the increased gluconeogenesis in the liver. Finally, glucose metabolism is suppressed by GH at several steps in the glycolytic pathway.
A major effect of GH on liver is to stimulate production and release of IGFs. The IGFs are also known as somatomedins. The two somatomedins in humans share structural homologies with proinsulin, and both have substantial insulin-like growth activity—hence the designations insulin-like growth factor I (human IGF-I, or somatomedin-C) and insulin-like growth factor II (human IGF-II, or somatomedin A). IGF-I is a single-chain basic peptide that has 70 amino acids, and IGF-II is slightly acidic, with 67 amino acids. These two peptides are identical to insulin in half of their residues. In addition, they contain a structural domain that is homologous to the C-peptide of proinsulin.
A broad spectrum of normal cells respond to high doses of insulin by increasing thymidine uptake and initiating cell propagation. In most instances, IGF-I causes the same response as insulin in these cells but at significantly lower, more physiologic concentrations. Thus, the IGFs are more potent than insulin in their growth-promoting actions.
Evidence suggests that the IGFs exert their effects through either an endocrine or a paracrine/autocrine mechanism. IGF-I appears to stimulate cell propagation and growth by binding to specific IGF-I receptors on the plasma membrane of target cells, rather than binding to GH receptors (Fig. 41.5).
FIGURE 41.5 Production and action of insulin-like growth factor (IGF). The hypothalamus produces growth hormone–releasing hormone (GHRH), which stimulates somatotrophs in the anterior pituitary to release growth hormone (GH). Growth hormone release–inhibiting hormone (GHRIH) inhibits GH release. GH binds to cell surface receptors and stimulates IGF production and release by liver and other tissues. IGF binds to cell surface receptors and stimulates the phosphorylation of proteins that lead to cell division and growth.
Like insulin, the intracellular portion of the plasma membrane receptor for IGF-I (but not IGF-II) has intrinsic tyrosine kinase activity. The fact that the receptors for insulin and a number of other growth factors have intrinsic tyrosine kinase activity indicates that tyrosine phosphorylation initiates the process of cellular replication and growth. Subsequently, a chain of kinases is activated, which include a number of proto-oncogene products (see Chapters 10 and 17).
C. Catecholamines (Epinephrine, Norepinephrine, Dopamine)
The catecholamines belong to a family of bioamines and are secretory products of the sympathoadrenal system. They are required for the body to adapt to a great variety of acute and chronic stresses. Epinephrine (80% to 85% of stored catecholamines) is synthesized primarily in the cells of the adrenal medulla, whereas norepinephrine (15% to 20% of stored catecholamines) is synthesized and stored not only in the adrenal medulla but also in various areas of the central nervous system (CNS) and in the nerve endings of the adrenergic nervous system. Dopamine, another catecholamine, acts primarily as a neurotransmitter and has little effect on fuel metabolism.
The first total chemical synthesis of epinephrine was accomplished by Stolz et al. in 1904. In 1950, Sutherland was the first to demonstrate that epinephrine (and glucagon) induces glycogenolysis. This marked the beginning of our understanding of the molecular mechanisms through which hormones act.
1. Synthesis of the Catecholamines
Tyrosine is the precursor of the catecholamines. The pathway for the biosynthesis of these molecules is described in Chapter 46.
2. Secretion of the Catecholamines
Secretion of epinephrine and norepinephrine from the adrenal medulla is stimulated by a variety of stresses, including pain, hemorrhage, exercise, hypoglycemia, and hypoxia. Release is mediated by stress-induced transmission of nerve impulses emanating from adrenergic nuclei in the hypothalamus. These impulses stimulate the release of the neurotransmitter acetylcholine from preganglionic neurons that innervate the adrenomedullary cells. Acetylcholine depolarizes the plasma membranes of these cells, allowing the rapid entry of extracellular calcium (Ca2+) into the cytosol. Ca2+ stimulates the synthesis and release of epinephrine and norepinephrine from the chromaffin granules into the extracellular space by exocytosis.
3. Physiological Effects of Epinephrine and Norepinephrine
The catecholamines act through two major types of receptors on the plasma membrane of target cells, the α-adrenergic and the β-adrenergic receptors (see Chapter 19, Section IV.C).
The actions of epinephrine and norepinephrine in the liver, the adipocyte, the skeletal muscle cell, and the α– and β-cells of the pancreas directly influence fuel metabolism (Fig. 41.6). These catecholamines are counterregulatory hormones that have metabolic effects directed toward mobilization of fuels from their storage sites for oxidation by cells to meet the increased energy requirements of acute and chronic stress. They simultaneously suppress insulin secretion, which ensures that fuel fluxes will continue in the direction of fuel utilization rather than storage as long as the stressful stimulus persists.
FIGURE 41.6 Effects of epinephrine on fuel metabolism and pancreatic endocrine function. Epinephrine (Epi) stimulates glycogen breakdown in muscle and liver, gluconeogenesis in liver, and lipolysis in adipose tissue. Epinephrine further reinforces these effects because it increases the secretion of glucagon, a hormone that shares many of the same effects as epinephrine. Epi also inhibits insulin release while stimulating glucagon release from the pancreas. FA, fatty acid; TG, triglyceride.
In addition, norepinephrine works as a neurotransmitter and affects the sympathetic nervous system in the heart, lungs, blood vessels, bladder, gut, and other organs. These effects of catecholamines on the heart and blood vessels increase cardiac output and systemic blood pressure, hemodynamic changes that facilitate the delivery of blood-borne fuels to metabolically active tissues.
Epinephrine has a short half-life in the blood and to be effective pharmacologically it must be administered parenterally. It may be used clinically to support the beating of the heart, to dilate inflamed bronchial muscles, and even to decrease bleeding from organs during surgery.
4. Metabolism and Inactivation of Catecholamines
Catecholamines have a relatively low affinity for both α- and β-receptors. After binding, the catecholamine disassociates from its receptor quickly, causing the duration of the biological response to be brief. The free hormone is degraded in several ways, as outlined in Chapter 46.
D. Glucocorticoids
1. Biochemistry
Cortisol (hydrocortisone) is the major physiological glucocorticoid (GC) in humans, although corticosterone also has some glucocorticoid activity. Glucocorticoids, such as cortisol, were named for their ability to raise blood glucose levels. These steroids are among the “counterregulatory” hormones that protect the body from insulin-induced hypoglycemia. The biosynthesis of steroid hormones and their basic mechanism of action has been described in Chapters 15 and 32.
2. Secretion of Glucocorticoids
The synthesis and secretion of cortisol are controlled by a cascade of neural and endocrine signals linked in tandem in the cerebrocortical–hypothalamic–pituitary–adrenocortical axis. Cerebrocortical signals to the midbrain are initiated in the cerebral cortex by “stressful” signals such as pain, hypoglycemia, hemorrhage, and exercise (Fig. 41.7). These nonspecific “stresses” elicit the production of monoamines in the cell bodies of neurons of the midbrain. Those that stimulate the synthesis and release of corticotropin-releasing hormone (CRH) are acetylcholine and serotonin. These neurotransmitters then induce the production of CRH by neurons originating in the paraventricular nucleus. These neurons discharge CRH into the hypothalamico-hypophyseal portal blood. CRH is delivered through these portal vessels to specific receptors on the cell membrane of the adrenocorticotropic hormone (ACTH)-secreting cells of the anterior pituitary gland (corticotrophs). This hormone–receptor interaction causes ACTH to be released into the general circulation, eventually to interact with specific receptors for ACTH on the plasma membranes of cells in the zona fasciculata and zona reticularus of the adrenal cortex. The major trophic influence of ACTH on cortisol synthesis is at the level of the conversion of cholesterol to pregnenolone, from which the adrenal steroid hormones are derived (see Chapter 32 for the biosynthesis of the steroid hormones).
FIGURE 41.7 Regulation of cortisol secretion. Various factors act on the hypothalamus to stimulate the release of corticotrophin-releasing hormone (CRH). CRH stimulates the release of adrenocorticotropic hormone (ACTH) from the anterior pituitary, which stimulates the release of cortisol from the adrenal cortex. Cortisol inhibits the release of CRH and ACTH via negative feedback loops.
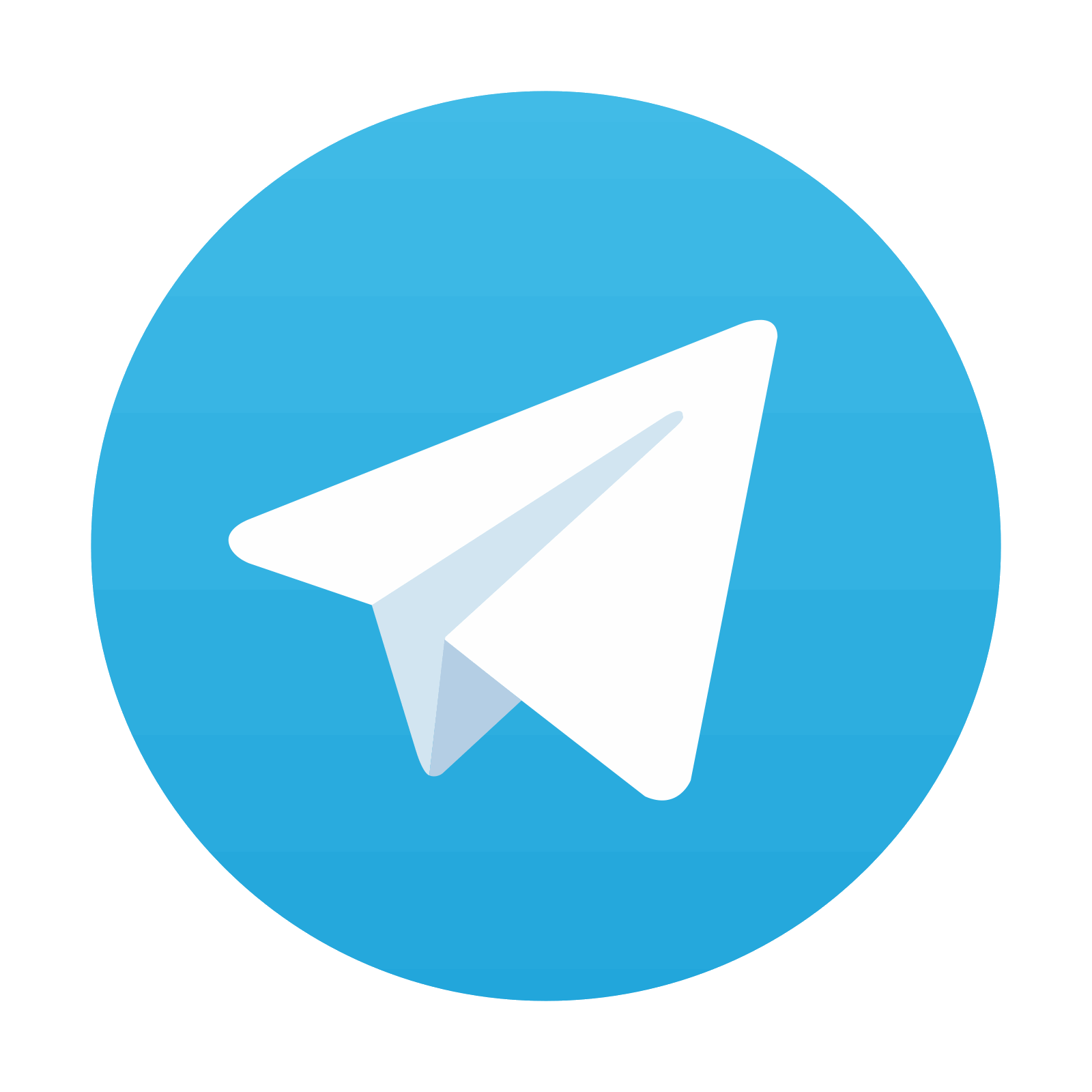
Stay updated, free articles. Join our Telegram channel
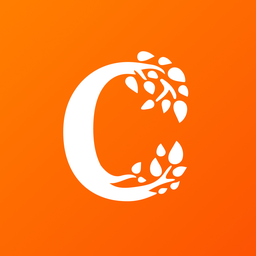
Full access? Get Clinical Tree
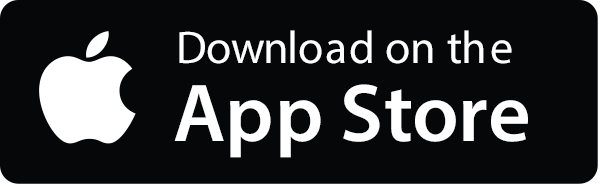
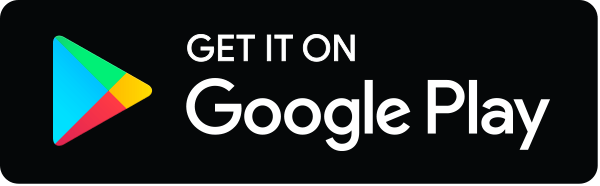