OBJECTIVES
After studying this chapter, you should be able to:
Describe the various parts of the eye and list the functions of each.
Describe the organization of the retina.
Explain how light rays in the environment are brought to a focus on the retina and the role of accommodation in this process.
Define hyperopia, myopia, astigmatism, presbyopia, and strabismus.
Describe the electrical responses produced by rods and cones, and explain how these responses are produced.
Describe the electrical responses and function of bipolar, horizontal, amacrine, and ganglion cells.
Trace the neural pathways that transmit visual information from the rods and cones to the visual cortex.
Describe the responses of cells in the visual cortex and the functional organization of the dorsal and ventral pathways to the parietal cortex.
Define and explain dark adaptation and visual acuity.
Describe the neural pathways involved in color vision.
Identify the muscles involved in eye movements.
Name the four types of eye movements and the function of each.
INTRODUCTION
The eyes are complex sense organs that have evolved from primitive light-sensitive spots on the surface of invertebrates. They gather information about the environment; and the brain interprets this information to form an image of what appears within the field of vision. The eye is often compared to a camera, with the cornea acting as the lens, the pupillary diameter functioning like the aperture of the camera, and the retina serving as the film. However, the eye, especially the retina, is far more sophisticated than even the most expensive camera. Within its protective casing, each eye has a layer of photoreceptors that respond to light, a lens system that focuses the light on these receptors, and a system of nerves that conducts impulses from the receptors to the brain. A great deal of work has been done on the neurophysiology of vision; in fact it is said to be the most studied and perhaps the best understood sensory system. The way the components of the visual system operate to set up conscious visual images is the subject of this chapter.
ANATOMY OF THE EYE
The principal structures of the eye are shown in Figure 9–1. The outer protective layer of the eyeball is the sclera or the “white of the eye” through which no light can pass. It is modified anteriorly to form the transparent cornea, through which light rays enter the eye. The lateral margin of the cornea is contiguous with the conjunctiva, a clear mucous membrane that covers the sclera. Just inside the sclera is the choroid, which is a vascular layer that provides oxygen and nutrients to the structures in the eye. Lining the posterior two-thirds of the choroid is the retina, the neural tissue containing the photoreceptors.
The crystalline lens is a transparent structure held in place by a circular lens suspensary ligament (zonule). The zonule is attached to the ciliary body, which contains circular muscle fibers and longitudinal muscle fibers that attach near the corneoscleral junction. In front of the lens is the pigmented and opaque iris, the colored portion of the eye. The iris, ciliary body, and choroid are collectively called the uvea. The iris contains circular muscle fibers that constrict and radial fibers that dilate the pupil. Variations in the diameter of the pupil can produce up to a fivefold change in the amount of light reaching the retina.
The aqueous humor is a clear protein-free liquid that nourishes the cornea and iris; it is produced in the ciliary body by diffusion and active transport from plasma. It flows through the pupil and fills the anterior chamber of the eye. It is normally reabsorbed through a network of trabeculae into the canal of Schlemm, which is a venous channel at the junction between the iris and the cornea (anterior chamber filtration angle). Obstruction of this outlet leads to increased intraocular pressure, a critical risk factor for glaucoma (Clinical Box 9–1).
The posterior chamber is a narrow aqueous-containing space between the iris, zonule, and the lens. The vitreous chamber is the space between the lens and the retina that is filled primarily with a clear gelatinous material called the vitreous (vitreous humor).
The eye is well protected from injury by the bony walls of the orbit. The cornea is moistened and kept clear by tears that course from the lacrimal gland in the upper portion of each orbit across the surface of the eye to empty via the lacrimal duct into the nose. Blinking helps keep the cornea moist.
The retina extends anteriorly almost to the ciliary body. It is organized into layers containing different types of cells and neural processes (Figure 9–2). The outer nuclear layer contains the photoreceptors, the rods, and the cones. The inner nuclear layer contains the cell bodies of the various types of excitatory and inhibitory interneurons including bipolar cells, horizontal cells, and amacrine cells. The ganglion cell layer contains the various types of ganglion cells that can be distinguished on the basis of morphology, projections, and functions. Ganglion cells are the only output neuron of the retina; their axons form the optic nerve. The outer plexiform layer is interposed between the outer and inner nuclear layers; the inner plexiform layer is interposed between the inner nuclear and ganglion cell layers. The neural elements of the retina are bound together by a type of glia called Müller cells, which form the inner limiting membrane, the boundary between the retina and the vitreous chamber. The elongated processes of these cells extend the entire thickness of the retina. The outer limiting membrane separates the inner segment portion of the rods and cones from their cell bodies.
FIGURE 9–2
Neural components of the extrafoveal portion of the retina. C, cone; R, rod; MB, RB, and FB, midget, rod, and flat bipolar cells; DG and MG, diffuse and midget ganglion cells; H, horizontal cells; A, amacrine cells. (Modified with permission from Dowling JE, Boycott BB: Organization of the primate retina: Electron microscopy, Proc R Soc Lond Ser B [Biol] 1966 Nov 15; 166(1002):80–111.)
The rods and cones, which are next to the choroid, synapse with bipolar cells, and the bipolar cells synapse with ganglion cells. There are various types of bipolar cells that differ in terms of morphology and function. Horizontal cells connect photoreceptor cells to the other photoreceptor cells in the outer plexiform layer. Amacrine cells connect ganglion cells to one another in the inner plexiform layer via processes of varying length and patterns. Amacrine cells also make connections on the terminals of bipolar cells. At least 29 types of amacrine cells have been described on the basis of their connections. Gap junctions also connect retinal neurons to one another.
CLINICAL BOX 9–1 Glaucoma
Increased intraocular pressure (IOP) is not the only cause of glaucoma, a degenerative disease in which there is loss of retinal ganglia cells; however, it is a critical risk factor. In 20–50% of the patients with this disease, IOP is normal (10–20 mm Hg); however, increased IOP makes glaucoma worse, and treatment is aimed at lowering the pressure. Indeed, elevations in IOP due to injury or surgery can cause glaucoma. Glaucoma is caused by poor drainage of the aqueous humor through the filtration angle formed between the iris and the cornea. Open-angle glaucoma, a chronic disease, is caused by decreased permeability through the trabeculae into the canal of Schlemm, which leads to an increase in IOP. In some cases, this type of glaucoma is due to a genetic defect. Closed-angle glaucoma results from a forward ballooning of the iris so that it reaches the back of the cornea and obliterates the filtration angle, thus reducing the outflow of aqueous humor. If left untreated, glaucoma can lead to blindness.
THERAPEUTIC HIGHLIGHTSGlaucoma can be treated with agents that decrease the secretion or production of aqueous humor or with drugs that increase outflow of the aqueous humor. β-Adrenergic blocking drugs such as timolol decrease the secretion of aqueous fluid. Carbonic anhydrase inhibitors (eg, dorzolamide, acetozolamide) also exert their beneficial effects by decreasing the secretion of aqueous humor. Glaucoma can also be treated with cholinergic agonists (eg, pilocarpine, carbachol, physostigmine) that increase aqueous outflow by causing ciliary muscle contraction. Aqueous outflow is also increased by prostaglandins. Prolonged use of corticosteroids can lead to glaucoma and increase the risk of occurrence of ocular infections due to fungi or viruses.
Because the receptor layer of the retina rests on the pigment epithelium next to the choroid, light rays must pass through the ganglion cell and bipolar cell layers to reach the rods and cones. The pigment epithelium absorbs light rays, preventing the reflection of rays back through the retina. Such reflection would otherwise produce blurring of the visual images.
The optic nerve leaves the eye at a point 3 mm medial to and slightly above the posterior pole of the globe. This region is visible through the ophthalmoscope as the optic disk (Figure 9–3). Since there are no visual receptors over the disk, this area of the retina does not respond to light and is known as the blind spot. Near the posterior pole of the eye, there is a yellowish pigmented spot called the macula. The fovea is in the center of the macula; it is a thinned-out, rod-free portion of the retina in humans and other primates. In it, the cones are densely packed, and each synapses on a single bipolar cell, which, in turn, synapses on a single ganglion cell, providing a direct pathway to the brain. There are very few overlying cells and no blood vessels. Consequently, the fovea is the point where visual acuity is greatest. When attention is attracted to or fixed on an object, the eyes are normally moved so that light rays coming from the object fall on the fovea. Age-related macular degeneration is a disease in which sharp, central vision is gradually destroyed (Clinical Box 9–2).
FIGURE 9–3
The fundus of the eye in a healthy human as seen through the ophthalmoscope. The fundus of the eye refers to the interior surface of the eye, opposite the lens, and includes the retina, optic disk, macula and fovea, and posterior pole. Optic nerve fibers leave the eyeball at the optic disk to form the optic nerve. The arteries, arterioles, and veins in the superficial layers of the retina near its vitreous surface can be seen through the ophthalmoscope. (Used with permission of Dr AJ Weber, Michigan State University.)
An ophthalmoscope is used to view the fundus of the eye, which is the interior surface of the eye, opposite to the lens; it includes the retina, optic disk, macula and fovea, and posterior pole (Figure 9–3). The arteries, arterioles, and veins in the superficial layers of the retina near its vitreous surface can be examined. Because this is the one place in the body where arterioles are readily visible, ophthalmoscopic examination is of great value in the diagnosis and evaluation of diabetes mellitus, hypertension, and other diseases that affect blood vessels. The retinal vessels supply the bipolar and ganglion cells, but the receptors are nourished, for the most part, by the capillary plexus in the choroid. This is why retinal detachment is so damaging to the receptor cells.
Glaucoma (Clinical Box 9–1) causes changes in the appearance of the fundus of the eye as seen through an ophthalmoscope (Figure 9–4). The photograph on the left is from a primate with a normal eye and shows an optic disk with a uniform “pinkish” color. The blood vessels appear relatively flat as they cross the margin of the disk. This is because there are a normal number of ganglion cell fibers, and the blood vessels have intact support tissue around them. The photograph on the right is from a primate with glaucoma that was experimentally induced by causing a chronic elevation in intraocular pressure. As is characteristic of glaucomatous optic neuropathy, the disk is pale, especially in the center. The retinal blood vessels are distorted, especially at the disk margin, due to a lack of support tissue; and there is increased “cupping” of the disk.
FIGURE 9–4
The fundus of the eye in a normal primate (left) and in a primate with experimentally induced glaucoma (right) as seen through an ophthalmoscope. Normal: uniform “pinkish” color vessels appear relatively flat crossing the margin of disk due to a normal number of ganglion cell fibers and since they have intact support tissue around them. Glaucomatous: disk is pale, especially in center, vessels are distorted, especially at the disk margin due to lack of support tissue and increased “cupping” of the disk. (Used with permission of Dr AJ Weber, Michigan State University.)
Each rod and cone photoreceptor is divided into an outer segment, an inner segment that includes a nuclear region, and a synaptic terminal zone (Figure 9–5). The outer segments are modified cilia composed of regular stacks of flattened saccules or membranous disks. The inner segments are rich in mitochondria; this is the region that synthesizes the photosensitive compounds. The inner and outer segments are connected by a ciliary stalk through which the photosensitive compounds travel from the inner segment to the outer segment of the rods and cones.
FIGURE 9–5
Schematic diagram of a rod and a cone. Each rod and cone is divided into an outer segment, an inner segment with a nuclear region, and a synaptic zone. The saccules and disks in the outer segment contain photosensitive compounds that react to light to initiate action potentials in the visual pathways. (Reproduced with permission from Lamb TD: Electrical responses of photoreceptors. In: Recent Advances in Physiology. No. 10. Baker PF [editor]. Churchill Livingstone, 1984.)
The rods are named for the thin, rodlike appearance of their outer segments. Each rod contains a stack of disk membranes that are flattened membrane-bound intracellular organelles that have detached from the outer membrane, and are thus free floating. Cones generally have thick inner segments and conical outer segments, although their morphology varies from place to place in the retina. The saccules of the cones are formed by infolding of the membrane of the outer segment. The saccules and disks contain the photosensitive compounds that react to light, initiating action potentials in the visual pathways.
Rod outer segments are being constantly renewed by the formation of new disks at the inner edge of the segment and phagocytosis of old disks from the outer tip by cells of the pigment epithelium. Cone renewal is a more diffused process and appears to occur at multiple sites in the outer segments.
In the extrafoveal portions of the retina, rods predominate (Figure 9–6), and there is a good deal of convergence. Flat bipolar cells (Figure 9–2) make synaptic contact with several cones, and rod bipolar cells make synaptic contact with several rods. Because there are approximately 6 million cones and 120 million rods in each human eye but only 1.2 million nerve fibers in each optic nerve, the overall convergence of receptors through bipolar cells on ganglion cells is about 105:1. However, there is divergence from this point on. For example, in the visual cortex the number of neurons concerned with vision is 1000 times the number of fibers in the optic nerves.
FIGURE 9–6
Rod and cone density along the horizontal meridian through the human retina. A plot of the relative acuity of vision in the various parts of the light-adapted eye would parallel the cone density curve; a similar plot of relative acuity of the dark-adapted eye would parallel the rod density curve.
CLINICAL BOX 9–2 Visual Acuity and Age-Related Macular Degeneration
Visual acuity is the degree to which the details and contours of objects are perceived, and it is usually defined in terms of the shortest distance by which two lines can be separated and still be perceived as two lines. Clinically, visual acuity is often determined by the use of the familiar Snellen letter charts viewed at a distance of 20 ft (6 m). The individual being tested reads aloud the smallest line distinguishable. The results are expressed as a fraction. The numerator of the fraction is 20, the distance at which the subject reads the chart. The denominator is the greatest distance from the chart at which a healthy individual can read the smallest line. Normal visual acuity is 20/20; a subject with 20/15 visual acuity has better than normal vision (not farsightedness); and one with 20/100 visual acuity has subnormal vision. Visual acuity is a complex phenomenon and is influenced by many factors, including optical factors (eg, the state of the image-forming mechanisms of the eye), retinal factors (eg, the state of the cones), and stimulus factors (eg, illumination, brightness of the stimulus, contrast between the stimulus and the background, length of time the subject is exposed to the stimulus). Many drugs can also have adverse side effects on visual acuity. Many patients treated with the antiarrhythmic drug amiodarone report corneal changes (kerotopathy) including complaints of blurred vision, glare and halos around lights, or light sensitivity. Aspirin and other anticoagulants can cause conjunctival or retinal hemorrhaging, which can impair vision. Maculopathy is a risk factor for those treated with tamoxifen for breast cancer. Antipsychotic therapies such as thioridazine can cause pigmentary changes, which can affect visual acuity, color vision, and dark adaptation.
There are over 20 million individuals in the United States and Europe with age-related macular degeneration (AMD), which is a deterioration of central visual acuity. Nearly 30% of those aged 75 or older have this disorder, and it is the most common cause of visual loss in those aged 50 or older. Women are at greater risk than men for AMD; also whites have a greater risk than blacks. There are two types: wet and dry. Wet AMD occurs when fragile blood vessels begin to form under the macula. Blood and fluid leak from these vessels and rapidly damage the macula. Vascular endothelial growth factors (VEGF) may contribute to the growth of these blood vessels. Dry AMD occurs when the cones in the macula slowly break down, causing a gradual loss of central vision.
THERAPEUTIC HIGHLIGHTSThe US Food and Drug Administration has approved ranibizumab (Lucentis) to treat wet AMD. It acts by inhibiting VEGF. Another drug approved for the treatment of wet AMD is pegaptanib sodium (Macugen), which attacks VEGF. Photodynamic therapy uses a drug called verteporfin, which is injected into the vein in an arm and is activated by a laser light, which produces a chemical reaction that destroys abnormal blood vessels. Laser surgery can be done to repair damaged blood vessels if they are at a distance from the fovea. However, new vessels may form after the surgery, and vision loss may progress.
One of the most important characteristics of the visual system is its ability to function over a wide range of light intensity. When one goes from near darkness to bright sunlight, light intensity increases by 10 log units, that is, by a factor of 10 billion. One factor reducing the fluctuation in intensity is adjustments in the diameter of the pupil; when the diameter is reduced from 8 to 2 mm, its area decreases by a factor of 16 and light intensity at the retina is reduced by more than 1 log unit.
Another factor in reacting to fluctuations in intensity is the presence of two types of photoreceptors. The rods are extremely sensitive to light and are the receptors for night vision (scotopic vision). The scotopic visual apparatus is incapable of resolving the details and boundaries of objects or determining their color. The cones have a much higher threshold, but the cone system has a much greater acuity and is the system responsible for vision in bright light (photopic vision) and for color vision. There are thus two kinds of inputs to the central nervous system (CNS) from the eye: input from the rods and input from the cones. The existence of these two kinds of input, each working maximally under different conditions of illumination, is called the duplicity theory.
THE PHOTORECEPTOR MECHANISM
The potential changes that initiate action potentials in the retina are generated by the action of light on photosensitive compounds in the rods and cones. When light is absorbed by these substances, their structure changes, and this triggers a sequence of events that initiates neural activity.
The eye is unique in that the receptor potentials of the photoreceptors and the electrical responses of most of the other neural elements in the retina are local, graded potentials, and it is only in the ganglion cells that all-or-none action potentials transmitted over appreciable distances are generated. The responses of the rods, cones, and horizontal cells are hyperpolarizing, and the responses of the bipolar cells are either hyperpolarizing or depolarizing, whereas amacrine cells produce depolarizing potentials and spikes that may act as generator potentials for the propagated spikes produced in the ganglion cells.
The cone receptor potential has a sharp onset and offset, whereas the rod receptor potential has a sharp onset and slow offset. The curves relating the amplitude of receptor potentials to stimulus intensity have similar shapes in rods and cones, but the rods are much more sensitive. Therefore, rod responses are proportional to stimulus intensity at levels of illumination that are below the threshold for cones. On the other hand, cone responses are proportional to stimulus intensity at high levels of illumination when the rod responses are maximal and cannot change. This is why cones generate good responses to changes in light intensity above background but do not represent absolute illumination well, whereas rods detect absolute illumination.
Na+ channels in the outer segments of the rods and cones are open in the dark, so current flows from the inner to the outer segment (Figure 9–7). Current also flows to the synaptic ending of the photoreceptor. The Na+, K+ ATPase in the inner segment maintains ionic equilibrium. Release of synaptic transmitter (glutamate) is steady in the dark. When light strikes the outer segment, the reactions that are initiated close some of the Na+ channels, and the result is a hyperpolarizing receptor potential. The hyperpolarization reduces the release of glutamate, and this generates a signal in the bipolar cells that ultimately leads to action potentials in ganglion cells. The action potentials are transmitted to the brain.
The photosensitive pigment in the rods is called rhodopsin (visual purple). Rhodopsin is composed of retinal, an aldehyde of vitamin A, and a protein called opsin. Because of the importance of vitamin A in the synthesis of retinal, it is not surprising that a deficiency in this vitamin produces visual abnormalities (Clinical Box 9–3).
Opsin has a molecular weight of 41 kDa. It is found in the membranes of the rod disks and makes up 90% of the total protein in these membranes. Opsin is part of the large family of G-protein-coupled receptors (GPCR). Retinal is parallel to the surface of the membrane (Figure 9–8) and is attached to a lysine residue at position 296 in the seventh transmembrane domain.
CLINICAL BOX 9–3 Vitamin A Deficiency
Vitamin A was the first fat-soluble vitamin identified and is composed of a family of compounds called retinoids. Deficiency is rare in the United States, but it is still a major public health problem in the developing world. Annually, about 80,000 individuals worldwide (mostly children in underdeveloped countries) lose their sight from severe vitamin A deficiency. Vitamin A deficiency is due to inadequate intake of foods high in vitamin A (liver, kidney, whole eggs, milk, cream, and cheese) or β-carotene, a precursor of vitamin A, found in dark green leafy vegetables and yellow or orange fruits and vegetables. One of the earliest visual defects to appear with vitamin A deficiency is night blindness (nyctalopia). Vitamin A deficiency also contributes to blindness by causing the eye to become very dry, which damages the cornea (xerophthalmia) and retina. Vitamin A first alters rod function, but concomitant cone degeneration occurs as vitamin A deficiency develops. Prolonged deficiency is associated with anatomic changes in the rods and cones followed by degeneration of the neural layers of the retina.
THERAPEUTIC HIGHLIGHTSTreatment with vitamin A can restore retinal function if given before the receptors are destroyed. Vitamin A–rich foods include liver, chicken, beef, eggs, whole milk, yams, carrots, spinach, kale, and other green vegetables. Other vitamins, especially those of the B complex, are also necessary for the normal functioning of the retina and other neural tissues.
The sequence of events in photoreceptors by which incident light leads to production of a signal in the next succeeding neural unit in the retina is summarized in Figure 9–9. In the dark, the retinal in rhodopsin is in the 11-cis configuration. The only action of light is to change the shape of the retinal, converting it to the all-trans isomer. This, in turn, alters the configuration of the opsin, and the opsin change activates its associated heterotrimeric G-protein, which in this case is called transducin, which has several subunits Tα, Gβ1, and Gγ1. After 11-cis retinal is converted to the all-trans configuration, it separates from the opsin in a process called bleaching. This changes the color from the rosy red of rhodopsin to the pale yellow of opsin.
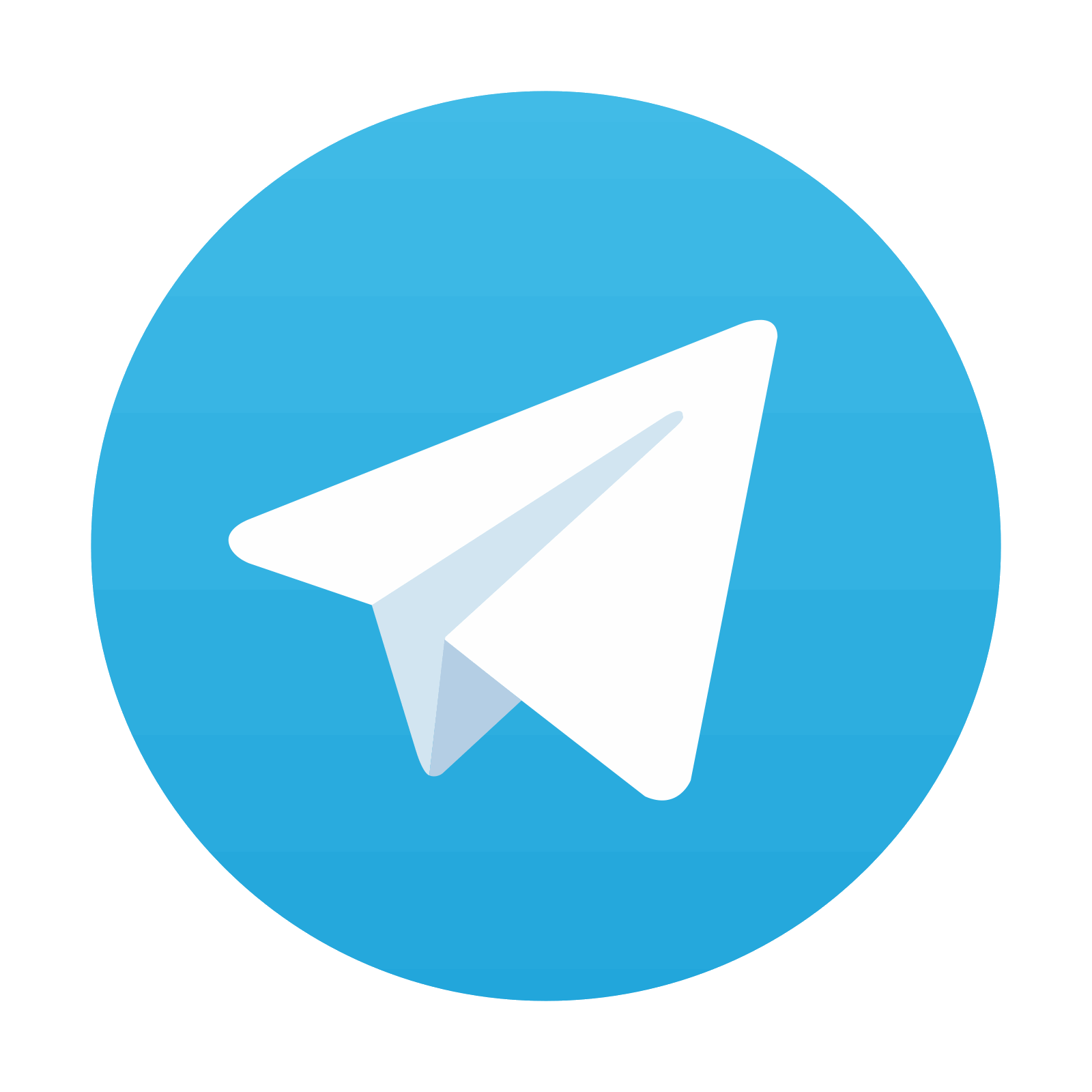
Stay updated, free articles. Join our Telegram channel
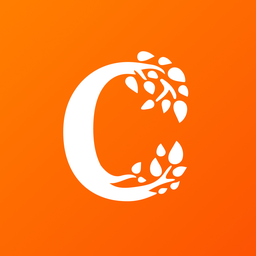
Full access? Get Clinical Tree
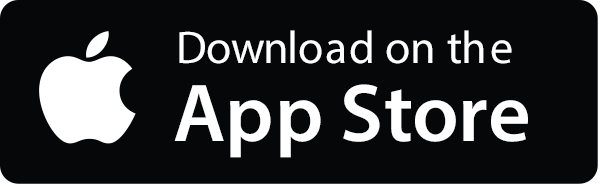
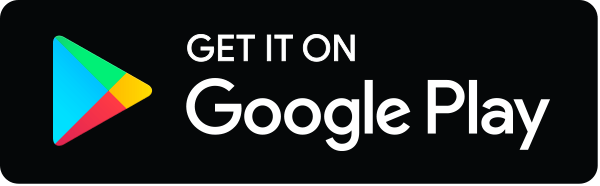