CHAPTER OUTLINE
O–Glycosylation and Mucin-Type O-Glycans
Hexosamine Biosynthesis Pathway
O-GlcNAcylation and Glucose Homeostasis
Lysosomal Targeting of Enzymes
Glycosylphosphatidylinositol-Anchored Proteins (GPI-Linkage)
Carbohydrate Recognition: Lectins
High-Yield Terms
Sugar code: refers to the total complement of sugars in an organism and how it relates to normal and abnormal physiology and pathology, also called the glycome
N-glycans: glycoprotein family with carbohydrate attachment to asparagine residues
O-glycans: glycoprotein family with carbohydrate attachment to serine, threonine, or hydroxylysine hydroxyl groups
Lipid-linked oligosaccharide (LLO): refers to the core glycan structure attached to the lipid dolichol pyrophosphate prior to addition to an asparagine residue of an N-linked glycoprotein; the core glycan structure is also referred to as the en bloc oligosaccharide
Hexosamine biosynthesis pathway (HBP): pathway for conversion of glucose to O-GlcNAc, which is important for the carbohydrate modification of numerous cytoplasmic and nuclear proteins
Lectin: any of a family of proteins that contain a carbohydrate-binding domain; lectin is derived from the Latin word meaning “to select”
Mucin: any of a family of high molecular weight, heavily glycosylated proteins with the ability to form gels that lubricate and form chemical barriers
Congenital disorders of glycosylation (CDG): a family of diseases resulting from inherited defects in the synthesis, processing, and modification of both N-linked and O-linked glycoproteins and related glycan-modified molecules
This significance underscores the relatively new field of study called glycomics, which analogous to genomics and proteomics, involves the analysis of the glycome (the total complement of sugars in an organism) and how it relates to normal and abnormal physiology and pathology. The glycome can be considered the sugar code of an organism.
Membrane-associated carbohydrate is exclusively in the form of oligosaccharides covalently attached to proteins, forming glycoproteins and to a lesser extent covalently attached to lipid, forming the glycolipids. Most proteins that are secreted, or bound to the plasma membrane, are modified by carbohydrate attachment. The posttranslational attachment of carbohydrate to proteins plays a critical role in overall biochemical complexity in humans. The importance of this protein modification can be emphasized by the fact that approximately 50% of all human proteins are known to be glycosylated and at least 1% of the human genome is represented by genes involved in glycan synthesis, processing, and homeostasis.
In addition to their critical roles in normal biochemical and physiological processes, the carbohydrate structures of many glycoproteins have been hijacked by pathogens and used as attachment sites for entry into host cells. HIV, poxviruses, parvoviruses, rhinoviruses, and herpes virus 6 all gain entry into cells by attaching to cell-surface glycoproteins. In addition, malarial parasite infection into erythrocytes involves recognition of cell-surface glycoproteins. Even bacteria utilize glycoproteins for attachment to host cells. Helicobacter pylori, the causative agent for one of the most common forms of cancer in humans, adenocarcinoma, adheres to gastric mucosal cells via glycoprotein recognition.
Glycoproteins
The predominant sugars found in glycoproteins are glucose (Glc), galactose (Gal), mannose (Man), fucose (Fuc), N-acetylgalactosamine (GalNAc), N-acetylglucosamine (GlcNAc), and N-acetylneuraminic acid (NANA). NANA is also called sialic acid (Sia) (Table 38-1). The distinction between proteoglycans (Chapter 39) and glycoproteins resides in the level and types of carbohydrate modification. Proteoglycans also contain the sugar glucuronic acid (GlcA) and the carbohydrate modifications found in glycoproteins are rarely as complex as that of proteoglycans.
The carbohydrates of glycoproteins are linked to the protein component through either O-glycosidic or N-glycosidic bonds (Figure 38-1). The N-glycosidic linkage is through the amide group of asparagine (N). In N-linked glycoproteins, the sugar attached to the N residue is always GlcNAc. The predominant carbohydrate attachment in glycoproteins of mammalian cells is via N-glycosidic linkage. The site of carbohydrate attachment to N-linked glycoproteins is found within a consensus sequence of amino acids, N-X-S/T (Asn-X-Ser/Thr), where X is any amino acid except Pro (P), Asp (D), or Glu (E). Analysis of the human protein database demonstrates that approximately 65% of all proteins contain at least one occurrence of the N-X-S/T consensus. The O-glycosidic linkage is to the hydroxyl of serine (S), threonine (T), or hydroxylysine (hLys). The linkage of carbohydrate to hLys is generally found only in the collagens. When attached to S or T, the sugar of O-linked glycoproteins is most often GalNAc. This most common O-glycoprotein type is also commonly referred to as a mucin-type glycan.
FIGURE 38-1: Representation of the N-glycosidic linkage of N-acetylglucosamine (GlcNAc) and the O-glycosidic linkage of N-acetylgalactosamine (GalNAc) to proteins. Reproduced with permission of themedicalbiochemistrypage, LLC.
The protein component of all glycoproteins is synthesized from polyribosomes that are bound to the ER. The processing of the sugar groups occurs co- and posttranslationally in the lumen of the ER and continues in the Golgi apparatus for N-linked glycoproteins. Attachment of sugars in O-linked glycoproteins occurs posttranslationally in the Golgi apparatus. Sugars used for glycoprotein synthesis (both N-linked and O-linked) are activated by coupling to nucleotides.
Nucleotide Sugar Biosynthesis
The monosaccharides that are attached to proteins are first activated by coupling to a nucleotide. The synthesis of nearly all nucleotide-activated sugars occurs in the cytosol. The exception to this is the synthesis of CMP-activated NANA, which takes place in the nucleus. Since the nucleotide-activated sugars are synthesized in the cytosol, they must be translocated into the ER and Golgi before they can be used in the process of glycan synthesis. Since nucleotide-activated sugars cannot freely pass through the ER or Golgi membranes, specific transport systems are responsible for their translocation. There are 2 mechanisms for the importation of nucleotide-activated sugars into the ER and/or Golgi apparatus. The first mechanism involves dolichol phosphate and it is used to transport mannose and glucose. The second mechanism involves specific nucleotide sugar transporters (NST) all of which belong to the solute carrier 35 (SLC35) family of membrane transporters. When the nucleotide-activated sugar is transported into the lumen of the ER or Golgi, there is a concomitant equimolar exit of a corresponding nucleoside monophosphate to the cytosol. Following entry into the ER or Golgi, a glycosyltransferase will transfer the monosaccharide to a target glycan concomitant with removal of the nucleoside diphosphate.
Mechanism of N-Glycosylation
Synthesis of carbohydrate portions of N-linked glycoproteins requires a lipid intermediate, dolichol phosphate (Figure 38-2). The phosphate moiety of dolichol phosphate is attached to the –OH group.
FIGURE 38-2: The structure of dolichol. The phosphate in dolichol phosphate is attached to the primary alcohol group at the left-hand end of the molecule. The group within the brackets is an isoprene unit (n = 17-20 isoprenoid units). Murray RK, Bender DA, Botham KM, Kennelly PJ, Rodwell VW, Weil PA. Harper’s Illustrated Biochemistry. 29th ed. New York, NY: McGraw-Hill; 2012.
Synthesis of the en bloc dolichol–PP–oligosaccharide unit (Figure 38-3) begins on the cytoplasmic face of the ER membrane and prior to transfer to the protein, the structure “flips” to the luminal side (Figure 38-4).
FIGURE 38-3: Structure of the en bloc oligosaccharide. Squares: GlcNAc; circles: mannose; triangles: glucose. Reproduced with permission of themedicalbiochemistrypage, LLC.
FIGURE 38-4: Synthesis and transfer of the LLO unit to a protein within the ER. The synthesis of the dolichol-coupled oligosaccharide complex (the lipid-linked oligosaccharide, LLO) begins on the cytosolic face of the ER membrane. When the dolichol-sugar complex contains the initial 2 GlcNAc residues and several mannose residues it is flipped such that the carbohydrate portion is moved to the luminal side of the ER membrane. Several more sugars are added to form the complete LLO. The sugars portion of the LLO is then transferred to an appropriate Asn residue in an acceptor protein, leaving the dolichol within the membrane. Reproduced with permission of themedicalbiochemistrypage, LLC.
Immediately following transfer of the en bloc oligosaccharide unit to the protein, processing and alteration of the composition of the oligosaccharide ensues and continues as the protein passes through the ER and then into and through the Golgi apparatus. Initially, the terminal glucose is removed through the action of glucosidase I (GI), a membrane-bound enzyme recognizing α-1,2-linked glucose. The remaining 2 glucose residues are then removed by glucosidase II (GII), a soluble enzyme recognizing α-1,3-linked glucose. After removal of the glucose residues, the action of α-mannosidases removes several mannose residues as the protein progresses to the Golgi. The action of the various glucosidases and mannosidases leaves N-linked glycoproteins containing a common core of carbohydrate consisting of 3 mannose residues and 2 GlcNAc. Through the action of a wide range of glycosyltransferases and glycosidases, a variety of other sugars are attached to this core as the protein progresses through the Golgi ultimately generating 3 major families of N-linked glycoproteins (Figure 38-5). A summary of the steps of N-glycosylation are presented in Table 38-2.
FIGURE 38-5: Structures of oligosaccharides found on the 3 major classes of N-glycoprotein. Open squares: GlcNAc; open circles: mannose; open diamonds: galactose; filled squares: fucose; filled triangles: sialic acid. Reproduced with permission of themedicalbiochemistrypage, LLC.
O-Glycosylation and Mucin-Type O-Glycans
The attachment of carbohydrate to the hydroxyl group of serine (Ser, S) or threonine (Thr, T) residues in proteins, as well as hydroxylysine (hLys) in collagens, constitutes the bulk of O-linked glycans (O-glycans) found in the humans. Carbohydrate addition in O-glycans occurs via the stepwise addition of nucleotide-activated sugars as the modified proteins traverse the ER and the Golgi network. There are 7 major types of O-glycans in humans that are defined by the first sugar residue that is attached to Ser, Thr, or hLys (Table 38-3).
High-Yield Concept
The synthesis of nucleotide-activated sugars is under tight regulatory control such that the alteration in production of only a single nucleotide sugar can significantly impair glycosylation with potentially profound effects. That the disruption in the processes of nucleotide-activated sugar synthesis can result in severe clinical symptomatology is evident from the large number of disorders classified as congenital disorders of glycosylation, CDG (Clinical Box 38-2).
The formation of the GlcNAc-β-N linkage in proteins occurs in the endoplasmic reticulum (ER) through cotranslational addition of a preassembled carbohydrate core structure that is delivered via the carbohydrate-dolichol intermediate. The structure is abbreviated as Glc3Man9GlcNAc2–PP–dolichol and is commonly referred to as the lipid-linked oligosaccharide (LLO) and the oligosaccharide structure itself is termed the en bloc oligosaccharide (Figure 38-3).
The process of O-GalNAc glycosylation occurs via 2 distinct steps that consist of initiation and processing. The initiation step controls the pattern and the density of the carbohydrate structures attached to the protein. The processing step determines the ultimate O-glycan structure that is present in the fully modified protein. The attachment of the initial GalNAc residue occurs in the Golgi network after the target protein has obtained its native folded state. Attachment of GalNAc to Ser or Thr residues in a target protein is catalyzed by a family of enzymes known as UDP-N-acetylgalactosamine: polypeptide N-acetylglucosaminyltransferases (ppGalNAcTs or ppGaNTases). To date, a total of 17 functionally active ppGaNTases have been characterized in humans. Numerous glycosyltransferases carry out the processes of modification of the carbohydrate structures resulting in the fully modified O-glycan. A summary of the steps of O-glycosylation are presented in Table 38-4.
Of the 7 different types of O-glycans, by far the most common in humans is the mucin type. Mucin glycoproteins are so called because of their abundance in the mucous secretions on cell surfaces and in body fluids. Mucin-type O-glycans all have the amino sugar GalNAc attached to the Ser or Thr residue of the modified protein. There are 8 mucin-type core structures, which are defined based upon the second sugar attached to the GalNAc and the linkage of that attachment.
The attachment of carbohydrates to proteins forming O-glycans serves multiple critical functions with respect to the structure and activity of the modified proteins. O-linked glycans are important for protein stability, modulation of enzyme activity, receptor-mediated signaling, immune function and immunity, protein-protein interactions, as well as many other functions. Mucin-type O-glycans are also important for binding water and are often found on the outer surfaces of tissues, such as the gastrointestinal, urogenital, and respiratory systems (Table 38-5).
O-Mannosylation
Incorporation of mannose into target proteins by attachment to Ser and/or Thr, referred to as O-mannosylation, serves a critical function in humans. O-mannosylation has been identified on α-dystroglycan (α-DG) from nerve and muscle, chondroitin sulfate proteoglycans, and several other proteins. Clinically, O-mannosylation of α-DG is the most significant as evidenced by the constellation of symptoms that result from defects in the processes of O-mannosylation.
Incorporation of mannose into O-glycans involves the transfer of GDP-activated mannose (GDP-Man) to dolichol-phosphate forming Dol-P-Man. Since it occurs in the ER, the actual process of O-mannosylation is distinct from most other O-glycosylation reactions that take place exclusively in the Golgi apparatus. After mannosylation of the target protein, further extension of the O-linked mannose residue takes place in the Golgi apparatus.
The best-studied O-mannosylated protein in humans is α-DG. It comprises 2 globular domains separated by a Ser/Thr-rich mucin-like region that is substantially O-mannosylated. α-DG is an essential component of the dystrophin-glycoprotein complex (DGC) in skeletal muscle. Highlighting the significance of O-mannosylated α-DG is the fact that most of the defects associated with impaired O-mannosylation can be explained by reduced function of α-DG. In humans, defective O-mannosylation is associated with a group of autosomal recessive muscular dystrophies termed congenital muscular dystrophies (CMDs). The CMDs are also referred to as secondary α-dystroglycanopathies since their common pathological feature is the hypoglycosylation of α-DG. Mutations in 6 glycosyltransferase genes have been identified to cause various α-dystroglycanopathies. The most severe CMD is Walker-Warburg syndrome (WWS) and as a consequence of multiple malformations, WWS patients often die within the first year of life.
Hexosamine Biosynthesis Pathway
Numerous nuclear and cytoplasmic proteins are posttranslationally modified on serine and threonine residues with β-N-acetylglucosamine (O-GlcNAc), in a process referred to as O-GlcNAcylation. The gamut of O-GlcNAcylated proteins includes enzymes involved in the metabolism of amino acids, nucleotides (eg, thymidine kinase), and carbohydrates (eg, glucose-6-phosphatase), general metabolic processes, cell growth and maintenance (eg, MYC, Sp1), DNA damage responses, intracellular transport, transcription (eg, RNA polymerase II), and translation (eg, eIF-5). The carboxy-terminal domain of a subpopulation of RNA polymerase II is extensively O-GlcNAcylated, and almost all RNA polymerase II transcription factors (TFII) are O-GlcNAcylated. Thus far, more than 600 proteins have been shown to be O-GlcNAcylated.
The synthesis of O-GlcNAc, which initiates with glucose, occurs via the metabolic pathway called the hexosamine biosynthesis pathway, HBP (Figure 38-6). Upon entering cells, most of the glucose is utilized for glycolysis or glycogen synthesis or in the pentose phosphate pathway; however, 2% to 5% will enter the HBP to generate uridine diphospho-N-acetylglucosamine (UDP-GlcNAc). The end product of the HBP, UDP-GlcNAc, is an essential intermediate in the synthesis of a wide array of complex glycans.
FIGURE 38-6: The hexosamine biosynthesis pathway (HBP). Although the majority of the glucose taken up by the cell is committed to glycolysis, glycogen synthesis, and the pentose phosphate pathway, in general about 2% to 5% of it enters the HBP for the formation of UDP-GlcNAc. The UDP-GlcNAc then serves as the substrate for a wide array of glycosylations, including O-GlcNAcylation. The first and rate-limiting enzyme in the HBP is glutamine:fructose 6-phosphate aminotransferase 1 (GFAT; also abbreviated GFPT1). Like other metabolic rate-limiting enzymes, GFAT is negatively regulated by the end product of the pathway, UDP-GlcNAc. Glucosamine can enter the HBP directly by conversion to GlcN6P via the action of hexokinase, thus bypassing the rate-limiting GFAT step. EMeg32 is glucosamine 6-phosphate N-acetyltransferase (also abbreviated GNPNAT1). The derivation EMeg32 is from the fact that the gene was originally isolated from a screen for genes specific for precursors of Erythroid and Megakaryocytic lineages and was clone 32. PHI is phosphohexose isomerase. PAGM is phosphoacetylglucosamine mutase. Reproduced with permission of themedicalbiochemistrypage, LLC.
Whereas there are hundreds of different kinases and phosphatases that add and remove phosphate from serine and threonine residues, respectively, in various target proteins, the process of O-GlcNAc cycling is maintained by the action of only 2 enzymes. Addition of GlcNAc (O-GlcNAcylation) is catalyzed by the enzyme UDP-N-acetylglucosamine:polypeptide β-N-acetylglucosaminyltransferase or more commonly just O-GlcNAc transferase, OGT. Removal of the O-GlcNAc modification is catalyzed by β-N-acetylglucosaminidase or more commonly O-GlcNAcase, OGA.
The HBP integrates the nutrient status of the cell by utilizing glucose, acetyl-CoA, glutamine, and UTP to produce UDP-GlcNAc. In turn, OGT will transmit this nutrient information throughout the cell changing the level of GlcNAcylated target proteins. This means that the cycling of O-GlcNAc is a nutrient-responsive, posttranslational modification that, like phosphorylation, impacts target protein activity. Additionally, disruption of the HBP has a profound impact on diseases of nutrient sensing, such as Type 2 diabetes. Numerous proteins involved in insulin signaling and the downstream targets of these signaling cascades have been shown to be O-GlcNAcylated. This modification of proteins involved in insulin signaling plays an important role in the development of insulin resistance under conditions of metabolic disruption (see Chapter 46).
High-Yield Concept
Unlike all other sugar modification of proteins, O – GlcNAc modification at serine and threonine residues cycles between addition and removal throughout the life of a target protein. In this respect O – GlcNAcylation is a modification akin to protein phosphorylation and dephosphorylation.
High-Yield Concept
Overexpression of the rate-limiting enzyme of the HBP, GFAT, leads to peripheral insulin resistance. In addition, overexpression of O-GlcNAc transferase (OGT) in skeletal muscle and adipose tissue results in elevated circulating insulin levels and insulin resistance.
O-GlcNAcylation and Glucose Homeostasis
Insulin resistance is a major contributing factor to the hyperglycemia typical of Type 2 diabetes, due in part, to the loss of GLUT4 presentation in the plasma membranes of skeletal muscle and adipose tissue. Aberrantly regulated hepatic gluconeogenesis also contributes to hyperglycemia. Several transcription factors (eg, FOXO1 and PGC-1α), that are involved in the regulated expression of gluconeogenic genes, can be modified by O-GlcNAcylation. The activity of OGT ultimately regulates gluconeogenesis through O-GlcNAcylation of PGC-1α that stabilizes the protein by inhibiting its ubiquitination. Stabilization of PGC-1α results in enhanced hepatic gluconeogenesis, allowing for more glucose to be delivered to the blood.
Lysosomal Targeting of Enzymes
Enzymes that are destined for the lysosomes (lysosomal enzymes) are directed there by a specific carbohydrate modification. During transit through the Golgi apparatus, a residue of GlcNAc-1-phosphate (GlcNAc-1-P) is added to the carbon-6 hydroxyl group of one or more specific mannose residues that have been added to these enzymes. The GlcNAc is transferred by UDP-GlcNAc:lysosomal enzyme GlcNAc-1-phosphotransferase (GlcNAc-phosphotransferase), yielding a phosphodiester intermediate: GlcNAc-1-P-6-Man-protein. The phosphotransferase is a hexameric complex whose protein subunits are encoded by 2 genes. The α- and β-subunits of the phosphotransferase are encoded by the GNPTAB gene. The second reaction (catalyzed by GlcNAc-1-phosphodiester-N-acetylglucosaminidase) removes the GlcNAc, leaving mannose residues phosphorylated in the 6 position: Man-6-P protein. A specific Man-6-P receptor (MPR) is present in the membranes of the Golgi apparatus. Binding of Man-6-P to this receptor targets proteins to the lysosomes. Defects in the process of mannose-6-phosphorylation result in several disorders referred to as mucolipidoses (eg, I-cell disease, Clinical Box 38-1).
Glycosylphosphatidylinositol-Anchored Proteins (GPI-Linkage)
Many membrane-associated glycoproteins are tethered to the outer leaflet of the plasma membrane via a glycosylphosphatidylinositol (GPI) linkage to their C-termini (Figure 38-7). These types of glycoproteins are termed glypiated proteins. The use of the GPI anchor has been found in a functionally diverse array of mammalian proteins that includes hydrolytic enzymes, adhesion molecules, complement regulatory proteins, and receptors. One clinically important glypiated protein is the erythrocyte surface glycoprotein, decay-accelerating factor (DAF; also known as CD55 = cluster of differentiation protein 55). DAF prevents erythrocyte lysis by complement. Other important GPI-linked proteins are the enzymes acetylcholinesterase, the cell adhesion molecule N-CAM-120 (neural cell adhesion molecule-120), and the T-cell markers Thy-1 and LFA-3 (lymphocyte function–associated antigen-3).
FIGURE 38-7: General structure of the GPI anchor. R1 is most often an unsaturated fatty acid but can be just –OH; R2 is either a fatty acid, alkyl or alkenyl group, can also be ceramide instead of glycerolipid, can also just be –OH; R3 is most often palmitic acid attached to the C–2 carbon of inositol; R4, R9 are ethanolamine phosphate or –OH; R5, R6, R7, R8, R10 are carbohydrate substituents or –OH. Reproduced with permission of themedicalbiochemistrypage, LLC.
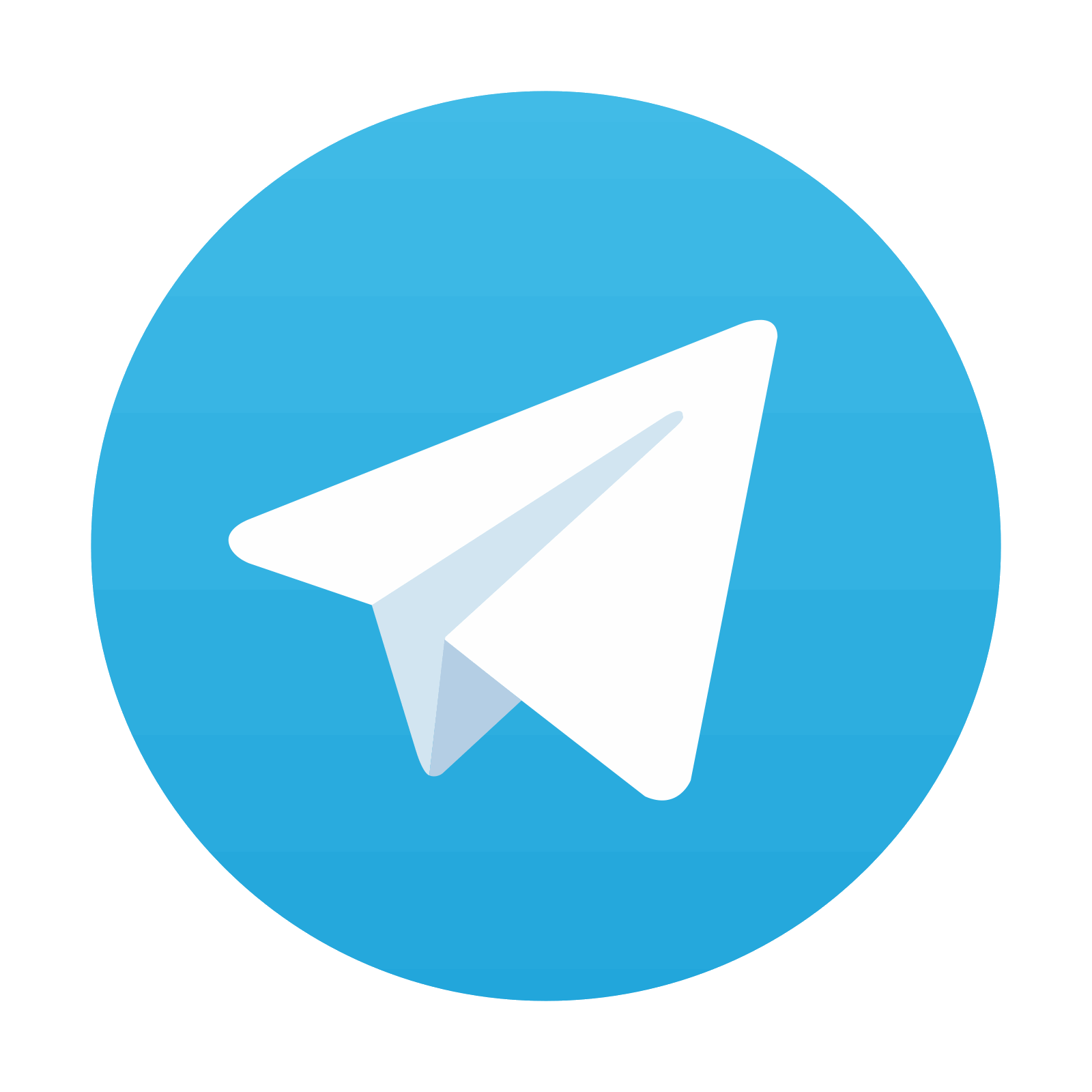
Stay updated, free articles. Join our Telegram channel
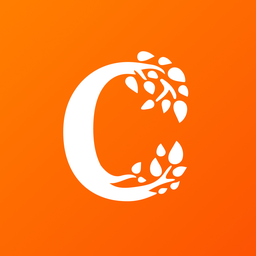
Full access? Get Clinical Tree
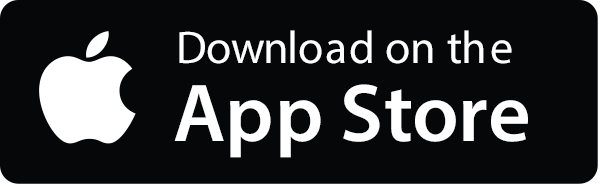
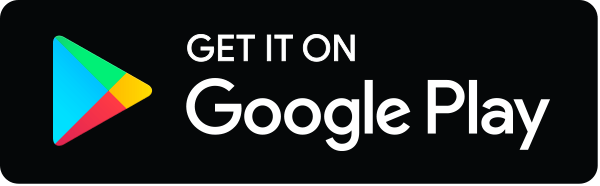