CHAPTER OUTLINE
Determination of the Genetic Code
Regulation of Translation Initiation: The eIF-2α Kinases
Iron-Mediated Control of Translation
Secreted and Membrane-Associated Proteins
Membrane Targeting by Prenylation
High-Yield Terms
Genetic code: the genetic code defines the set of rules (the nucleotide triplets) by which information encoded within the genes is translated into proteins
Wobble hypothesis: explains the non–Watson-Crick base-pairing that can occur between the 3′-nucleotide of a codon and the 5′-nucleotide of an anticodon
Ternary complex: translational initiation complex composed of eIF-2 with GTP bound and the initiator methionine tRNA
Peptidyltransferase: an RNA-mediated (ribozyme) enzymatic activity of the 60S ribosome that catalyzes transpeptidation during protein elongation.
Selenoprotein: any of a family of proteins that contain a modified selenocysteine amino acid
Signal sequence: the N-terminal 15-25 hydrophobic amino acids in proteins that are destined for secretion of membrane insertion; the sequences are recognized by the signal recognition particle which assists in binding of the ribosome to the ER membrane
Prenylation: posttranslational modification by the isoprenoid molecules farnesyl or geranyl allowing proteins to associate with membranes
Ubiquitin: a 76-amino-acid peptide enzymatically attached to proteins which, in most cases, targets the modified protein for degradation in the proteosome
Proteosome: a large protein complex whose function is to proteolytically degrade damaged or unneeded proteins
Translation is the RNA-directed synthesis of proteins. The process requires not only the template RNA, the mRNA, but also the participation of the tRNAs and rRNAs. The tRNAs are necessary to carry activated amino acids into the ribosome which itself is composed of rRNAs and ribosomal proteins. Although the chemistry of peptide bond formation is relatively simple, the processes leading to the ability to form a peptide bond are exceedingly complex. During translation the ribosome is intimately associated with the mRNA ensuring correct access of activated tRNAs and the necessary enzymatic activities to catalyze peptide bond formation.
Translation proceeds in an ordered process. First, accurate and efficient initiation must take place, then peptide elongation, and finally accurate and efficient termination must occur. All three of these processes require specific proteins, some of which are ribosome associated and some of which are separate from the ribosome, but may be temporarily associated with it.
Determination of the Genetic Code
Early experiments, designed to ascertain how the information in the DNA of genes was transmitted via RNAs to proteins, demonstrated several key facts that resulted in the definition of the genetic code. In addition, it was determined that the genetic code is read in a sequential manner starting from a fixed point in the mRNA and that the 5′-end of the mRNA corresponded to the amino terminus of the encoded protein. This means that translation proceeds along the mRNA in the 5′ → 3′ direction which corresponds to the N-terminal to C-terminal direction of the amino acid sequences within proteins.
The code was shown to be a triplet of nucleotides and all 64 possible combinations of the 4 nucleotides could code for amino acids. This latter fact is defined as the degeneracy of the genetic code since there are only 20 different amino acids required for the synthesis of all the different proteins. The precise dictionary of the genetic code was originally determined from in vitro experiments which also established the identity of translational termination codons (Table 37-1).
In addition to the genetic code present in the mRNA, accurate translation requires 2 equally important recognition steps. The correct choice of amino acid needs to be made for attachment to the correspondingly correct tRNA and the subsequent selection of the correct amino acid–charged tRNA by the translational machinery so that the amino acid is incorporated in the correct location in the protein.
Characteristics of tRNAs
More than 300 different tRNAs have been characterized and shown to have extensive similarities. They vary in length from 60 to 95 nucleotides (18-28 kD) and exhibit a cloverleaf-like secondary structure that is composed of an anticodon loop, a D-loop, and a TψC-loop (Figure 37-1). The D-loop contains the modified nucleotide, dihydrouridine, and the TΨC loop contains pseudouridine, hence the derivation of the names for these loops. All tRNAs also have the trinucleotide sequence, CCA, added to their 3′-ends posttranscriptionally. The A residue serves as the acceptor site for attachment of the appropriate amino acid.
FIGURE 37-1: Two-dimensional structure of a typical tRNA. Reproduced with permission of themedicalbiochemistrypage, LLC.
Amino Acid Activation
Activation of amino acids is carried out by a 2-step process catalyzed by aminoacyl-tRNA synthetases (Figure 37-2). There are at least 21 different aminoacyl-tRNA synthetases since the initiator met-tRNA is distinct from noninitiator met-tRNAs. Accurate recognition of the correct amino acid as well as the correct tRNA is different for each aminoacyl-tRNA synthetase.
FIGURE 37-2: Formation of aminoacyl-tRNA. A 2-step reaction involving the enzyme amino-acyl-tRNA synthetase results in the formation of aminoacyl-tRNA. The first reaction involves the formation of an AMP-amino acid–enzyme complex. This activated amino acid is next transferred to the corresponding tRNA molecule. The AMP and enzyme are released, and the latter can be reutilized. The charging reactions have an error rate (ie, esterifying the incorrect amino acid on tRNAx) of less than 10−4. Murray RK, Bender DA, Botham KM, Kennelly PJ, Rodwell VW, Weil PA. Harper’s Illustrated Biochemistry. 29th ed. New York: McGraw-Hill; 2012.
In the 2-step reaction the enzyme first attaches the amino acid to the α-phosphate of ATP with the concomitant release of pyrophosphate forming an aminoacyl-adenylate intermediate. In the second step the enzyme catalyzes transfer of the amino acid to either the 2′–or 3′–OH of the ribose portion of the 3′-terminal adenosine residue of the tRNA generating the activated aminoacyl-tRNA.
Most cells contain isoacceptor tRNAs, different tRNAs that are specific for the same amino acid; however, many tRNAs bind to 2 or 3 codons specifying their cognate amino acids (see Table 37-1). Given the high degree of nucleotide modifications that occur in tRNAs it is possible for non–Watson-Crick base-pairing to occur at the third codon position, that is, the 3′-nucleotide of the mRNA codon and the 5′-nucleotide of the tRNA anticodon. This phenomenon has been termed the wobble hypothesis.
Translation Initiation
Initiation of translation requires numerous components including an mRNA, a pool of amino acid charged tRNAs including a specific initiator tRNA, tRNAimet, the ribosome, and numerous nonribosomal proteins termed initiation factors (Table 37-2). Translational initiation factors are identified as eIFs (eukar yotic translation initiation factors).
Initiation of translation requires four specific steps: 1) a ribosome must dissociate into its’ 40S and 60S subunits; 2) a ternary complex (termed the pre-initiation complex) consisting of the initiator met-tRNA, eIF-2 and GTP must engage the dissociated 40S subunit; 3) the mRNA is then bound to the pre-initiation complex; 4) the 60S subunit must associate with the pre-initiation complex to form the 80S initiation complex (Figure 37-3).
FIGURE 37-3: Details of the complex processes required for accurate and efficient initiation of translation. Several initiation factors (eg, eIF-1 and eIF-3) are required to ensure that the 60S and 40S ribosomal subunits remain separated (antiassociation) so that new rounds of translation can begin. The ternary complex, composed of GTP bound to the α-subunit of eIF2 and the initiator methionyl-tRNAmet, can engage the 40S subunit. The eIF-4F complex, which comprises the cap-binding factor, eIF-4E, the RNA helicase eIF-4A, and the scaffold subunit, eIF-4G, captures an mRNA and brings it to the 40S subunit and the ternary complex. Once the mRNA, 40S and 60S subunits, and the ternary complex are integrated, the resulting 80S initiation complex constitutes the completion of the process of translation initiation. PABP, polyA-binding protein. Reproduced with permission of themedicalbiochemistrypage, LLC.
It is absolutely necessary that the discrimination of correct amino acid and correct tRNA be made by a given synthetase prior to release of the aminoacyl-tRNA from the enzyme. Once the product is released there is no further way to proof-read whether a given tRNA is coupled to its correspondingly correct tRNA.
The cap structure of eukaryotic mRNAs is bound by eIF-4F prior to association with the preinitiation complex. This factor is actually a complex of 3 proteins; eIF-4E, eIF-4A, and eIF-4G. The protein eIF-4E physically recognizes and binds to the cap structure, eIF-4A binds and hydrolyzes ATP and exhibits RNA helicase activity to unwind mRNA secondary structure, and eIF-4G aids in binding of the mRNA to the 43S preinitiation complex.
The first step in the formation of the preinitiation complex is the binding of GTP to eIF-2 to form a binary complex. The binary complex then binds to the activated initiator tRNA, met-tRNAimet forming a ternary complex that then binds to the 40S subunit forming the 43S preinitiation complex.
Once the mRNA is properly aligned onto the preinitiation complex and the initiator met-tRNAimet is bound to the initiator AUG codon (a process facilitated by eIF-1), the 60S subunit associates with the complex forming the 80S initiation complex. The energy needed to stimulate the formation of the 80S initiation complex comes from the hydrolysis of the GTP bound to eIF-2. The large ribosomal subunit contains domains termed the A-site, P-site, and E-site. The A-site resides over the next codon to be recognized in the mRNA and each incoming aminoacyl-tRNA enters the ribosome at this site. The P-site is where the elongating protein attached to a tRNA will reside following each round of elongation. The E-site is where the protein is ejected from the ribosome upon completion of translation.
The GDP bound form of eIF-2 then binds to eIF-2B which stimulates the exchange of GTP for GDP on eIF-2. The overall process of eIF-2B–mediated GTP for GDP exchange in eIF-2 is termed the eIF-2 cycle (see Figure 37-3). The activity called eIF-2B is actually a complex of 5 subunits identified as α, β, γ, δ, and ε, each encoded by a separate gene. Inherited mutations in any one of these 5 genes results in a severe neurodegenerative disorder termed leukoencephalopathy with vanishing white matter (Clinical Box 37-1).
CLINICAL BOX 37-1: eIF-2B MUTATIONS AND DISEASE
Leukoencephalopathy with vanishing white matter (VWM), also referred to as childhood ataxia with central hypomyelination and myelinopathia centralis diffusa, is one of the most prevalent inherited white matter disorders of childhood but it can affect people of all ages, including neonates and adults. Although other tissues are affected, VWM is primarily a brain disorder in which oligodendrocytes and astrocytes are selectively affected. VWM is a progressive disorder clinically dominated by cerebellar ataxia and in which minor stress conditions, such as fever or mild trauma, can induce major episodes of neurological deterioration. Typical pathological findings are diminishing white matter, the gross pathology that lends its name to this disorder. Additional pathology includes cystic degeneration, oligodendrocytosis with highly characteristic foamy oligodendrocytes, limited astrogliosis with dysmorphic astrocytes, and apoptotic loss of oligodendrocytes. VWM is caused by mutations in any of the 5 genes (EIF2B1-EIF2B5), encoding the subunits (α, β, γ, δ, and ε) of initiation factor 2B (elF-2B). All identified mutations in these genes result in eIF-2B complexes with diminished activity and impair its function to couple protein synthesis to the cellular demands in basal conditions and during stress. The reduction in elF-2B function results in a sustained inappropriate activation of the unfolded protein response (UPR). Sustained activation of the UPR triggers an apoptotic process leading to the observed apoptotic loss of oligodendrocytes.
Regulation of Translation Initiation: The eIF-2α Kinases
Regulation of initiation in eukaryotes is primarily affected by phosphorylation of Ser (S) residues in the α-subunit of eIF-2 (eIF-2α). Phosphorylated eIF-2, in the absence of eIF-2B, is just as active an initiator as nonphosphorylated eIF-2. However, when eIF-2 is phosphorylated the GDP-bound complex is stabilized and exchange for GTP is inhibited. Regulation of translational initiation, via phosphorylation of eIF-2α, occurs under a number of different conditions that include endoplasmic reticulum (ER) stress, nutrient stress (deprivation or restriction), viral infection, and in erythrocytes as a consequence of limiting heme. Each of these distinct regulatory pathways involves a unique eIF-2α kinase, and there are 4 of these related kinases known to exist in mammalian cells (Figure 37-4).
FIGURE 37-4: Each of the various eIF-2α kinases responds to different physiological and/or environmental stressors and induces the phosphorylation of the α-subunit of eIF-2. In the case of nutritional stress or the unfolded protein response (UPR), the phosphorylation of eIF-2α results in the preferential translation of transcription factors (eg, ATF4) that initiate a pattern of gene expression that allows the cell to respond to the stress or in the case of severe and damaging stress the apoptotic program is triggered. GADD34 is a regulatory subunit of protein phosphatase-1 (PP-1). Transcription of the GADD34 gene by ATF4 allows for a feedback control loop on the level of eIF-2α phosphorylation. Reproduced with permission of themedicalbiochemistrypage, LLC.
The first eIF-2α kinase identified and characterized was isolated from reticulocyte lysates and shown to be involved in the control of globin mRNA translation in response to deficiency in heme. This kinase is called the heme-regulated inhibitor (HRI). When heme levels are low HRI is active and phosphorylates eIF-2α to limit translational initiation until there is adequate heme to generate functional hemoglobin. When heme levels rise eIF-2α is protected from phosphorylation by a protein that associates with the γ-subunit of eIF-2. Removal of the phosphate from eIF-2α is catalyzed by a specific eIF-2 phosphatase, which is unaffected by heme.
Viral infection involving double-stranded RNA viruses activates the expression of the interferons which in turn activate the eIF-2α kinase known as PKR (RNA-dependent protein kinase). Proteins that are destined for secretory vesicles are translated while associated with endoplasmic reticulum (ER) membranes.
During translation secreted proteins are transported into the lumen of the ER in an unfolded state. Proper folding occurs prior to the transfer of the protein to the Golgi apparatus. Under certain conditions the secretory responses of a cell can exceed the capacity of the folding processes of the ER. Accumulation of unfolded proteins triggers the unfolded protein response (UPR) which is a form of ER stress. The UPR activates an eIF-2α kinase termed PERK [RNA-dependent protein kinase (PKR)-like ER kinase]. Deficiencies in PERK result in the extremely rare autosomal recessive disorder known as Wolcott-Rallison syndrome, WRS (Clinical Box 37-2).
Nutritional deprivation, in particular amino acid deficiency, results in the activation of the eIF-2α kinase known as GCN2 (mammalian homolog of the general control nonderepressible-2 gene). In addition to nutritional deprivation, GCN2 is induced by UV irradiation, inhibition of proteosome function, and infection by certain viruses.
Regulation of eIF-4E Activity
At least 3 distinct mechanisms are known to exist that regulate the level and activity of eIF-4E. These include regulation of the level of transcription of the eIF-4E gene, posttranslational modification via phosphorylation, and inhibition by interaction with binding proteins. Although the exact effect of eIF-4E phosphorylation is not clearly defined, it may be necessary to increase affinity of eIF-4E for the mRNA cap structure and for eIF-4G. Of clinical significance is the observation that promiscuous elevation in the levels of eIF-4E can lead to tumorigenesis placing this translation factor in the category of a proto-oncogene.
CLINICAL BOX 37-2: WOLCOTT-RALLINSON SYNDROME
Wolcott-Rallison syndrome (WRS) is a rare autosomal recessive disease, characterized by neonatal/early-onset nonautoimmune insulin-requiring diabetes associated with skeletal dysplasia and growth retardation. WRS is the most frequent cause of neonatal/early-onset diabetes in patients with consanguineous parents. WRS should be suspected as the cause of diabetes mellitus occurring neonatally or before 6 months of age and originating from a population where there is a high prevalence of consanguinity. Additional signs in these children are skeletal dysplasia and/or episodes of acute liver failure. Other symptoms show variability in their nature and severity between patients with WRS. These additional symptoms include frequent episodes of acute liver failure, renal dysfunction, exocrine pancreas insufficiency, intellectual deficit, hypothyroidism, neutropenia, and recurrent infections. WRS is caused by mutations in the gene encoding the eIF-2α kinase, PERK (PKR-like endoplasmic reticulum kinase). PERK plays a key role in translation control during the unfolded protein response (UPR). Indeed, ER dysfunction is central to the disease processes in WRS. Molecular genetic testing confirms the diagnosis in patients suspected of having WRS. Aggressive therapeutic monitoring and interventional treatment of the diabetes with an insulin pump is highly recommended because of the risk of acute episodes of hypoglycemia and ketoacidosis. The prognosis for WRS patients is poor and most patients die at a young age.
The principal mechanism utilized in the regulation of eIF-4E activity is through its interaction with a family of binding/repressor proteins termed 4E-BPs (4E-binding proteins). Binding of 4E-BPs to eIF-4E does not alter the affinity of eIF-4E for the cap structure but prevents the interaction of eIF-4E with eIF-4G which in turn suppresses the formation of the eIF-4F complex. The ability of 4E-BPs to interact with eIF-4E is controlled via their state of phosphorylation (Figure 37-5). When not phosphorylated, 4E-BP binds with high efficiency to eIF-4E but lose their binding capacity when phosphorylated. Numerous growth factors, such as insulin, lead to phosphorylation of 4E-BPs, thereby releasing eIF-4E to interact efficiently with the cap structure allowing for increased translational initiation.
FIGURE 37-5: Activation of eIF-4E by insulin and its subsequent incorporation into the functional eIF-4F cap-binding complex. When 4E-BP is phosphorylated by insulin (as well as numerous other growth factors and mitogens), it dissociates from eIF-4E which allows the latter factor to interact with eIF-4G and then form the eIF-4F complex. Growth factors also induce phosphorylation of eIF-4G, generating a phosphorylated eIF-4F complex that interacts with the cap with higher affinity than nonphosphorylated eIF-4F. Murray RK, Bender DA, Botham KM, Kennelly PJ, Rodwell VW, Weil PA. Harper’s Illustrated Biochemistry. 29th ed. New York: McGraw-Hill; 2012.
Translation Elongation
The process of elongation like that of initiation requires specific nonribosomal proteins termed eEFs (elongation factors). Protein elongation occurs in a cyclic manner such that at the end of one complete round of amino acid addition the A-site will be empty and ready to accept the next incoming aminoacyl-tRNA dictated by the next codon of the mRNA (Figure 37-6). Each incoming aminoacyl-tRNA is brought to the ribosome by an eEF-1α-GTP complex. When the correct tRNA is deposited into the A-site, the GTP is hydrolyzed and the eEF-1α-GDP complex dissociates. In order for additional translocation events to occur, the GDP must be exchanged for GTP. This is carried out by eEF-1βγ similarly to the GTP exchange that occurs with eIF-2 catalyzed by eIF-2B.
FIGURE 37-6: The cyclical steps of elongation. The elongation factor eEF-1α carries each incoming aminoacyl-tRNA to the A-site. The peptide in the P-site is transferred to the amino acid in the A-site through the action of peptidyltransferase. Following peptide transfer the elongation factor eEF-2 induces translocation of the ribosome along the mRNA such that the naked tRNA is temporarily within the E-site and the peptidyl-tRNA is moved to the P-site leaving the A-site empty and residing over the next codon of the mRNA. Reproduced with permission of themedicalbiochemistrypage, LLC.
The peptide attached to the tRNA in the P-site is transferred to the amino acid on the aminoacyl-tRNA in the A-site forming a new peptide bond. This reaction is catalyzed by peptidyltransferase activity which resides in what is termed the peptidyltransferase center (PTC) of the large ribosomal subunit (60S subunit). This enzymatic process is termed transpeptidation. This enzymatic reaction is not mediated by any ribosomal proteins but instead by a ribozyme (see Chapter 36) contained in the 60S subunit.
The elongated peptide now resides on a tRNA in the A-site. The process of moving the peptidyl-tRNA from the A-site to the P-site is termed translocation and is catalyzed by eEF-2 coupled to GTP hydrolysis. In the process of translocation the ribosome is moved along the mRNA such that the next codon of the mRNA resides under the A-site. Following translocation eEF-2 is released from the ribosome.
The ability of eEF-2 to carry out translocation is regulated by the state of phosphorylation of the enzyme; when phosphorylated the eEF-2 activity is inhibited. Phosphorylation of eEF-2 is catalyzed by the enzyme eEF-2 kinase (eEF2K). Regulation of eEF2K activity is normally under the control of insulin and Ca2+ fluxes. The Ca2+-mediated effects are the result of calmodulin interaction with eEF2K. Activation of eEF2K in skeletal muscle by Ca2+ is important to reduce consumption of ATP in the process of protein synthesis during periods of exertion.
Translation Termination
Unlike initiation and elongation which require multiple protein activities, translational termination requires only 1 factor, termed eRF (for releasing factor). The signals for translation termination are the 3 termination codons, UAG, UAA, and UGA. The eRF binds to the A-site of the ribosome in conjunction with GTP (Figure 37-7). The binding of eRF to the ribosome stimulates the peptidyltransferase activity to transfer the peptidyl group to water instead of an aminoacyl-tRNA. The resulting uncharged tRNA left in the P-site is expelled with concomitant hydrolysis of GTP. The inactive ribosome then releases its mRNA and the 80S complex dissociates into the 40S and 60S subunits ready for another round of translation.
FIGURE 37-7: Translation termination occurs when a stop codon is encountered. The termination factor eRF, which binds GTP, then stimulates the peptidyltransferase to transfer the peptide, from the tRNA in the P-site to H2O coupled with the hydrolysis of GTP. The peptide is released from the ribosome along with the naked tRNA and eRF-GDP complex. Reproduced with permission of themedicalbiochemistrypage, LLC.
Selenoproteins
Selenium is a trace element and is found as a component of several enzymes that are involved in redox reactions such as glutathione peroxidase. The selenium in these selenoproteins is incorporated as a unique amino acid, selenocysteine, during translation. Selenocysteine incorporation in eukaryotic proteins occurs cotranslationally at UGA codons (normally stop codons) via the interactions of a number of specialized proteins and protein complexes (Figure 37-8). In addition, there are specific secondary structures in the 3′-untranslated regions (UTR) of selenoprotein mRNAs, termed SECIS elements that are required for selenocysteine insertion into the elongating protein. One of the complexes required for this important modification is comprised of a selenocysteinyl-tRNA [(Sec)-tRNA(Ser)Sec] and its specific elongation factor identified as selenoprotein translation factor B (SelB).
FIGURE 37-8: Selenocysteine biosynthesis and incorporation. The first steps involve the activation of serine onto the (Sec)-tRNA followed by enzymatic conversion to selenocysteine generating (Sec)-tRNA(Ser)Sec. Next the (Sec)-tRNA(Ser)Sec is bound by SelB and the complex is incorporated into the translational machinery. The elongating protein is transferred to the selenocysteinyl-tRNA via the action of peptidyltransferase as for any other incoming amino acid and normal elongation continues. Reproduced with permission of themedicalbiochemistrypage, LLC.
Iron-Mediated Control of Translation
Regulation of the translation of certain mRNAs occurs through the action of specific RNA-binding proteins. Proteins of this class have been identified as those that bind to sequences in either 5′-UTR or 3′-UTR. Regulation of iron homeostasis (Chapter 42) involves RNA binding proteins that bind to the transferrin receptor and ferritin mRNAs (Figure 37-9). When iron levels are low the rate of synthesis of the transferrin receptor increases so that cells can take up more iron, whereas translation of the ferritin mRNA is inhibited in order to restrict intracellular storage of iron. This regulation occurs through the action of an iron-response element–binding protein (IRBP) that binds to specific iron-response elements (IRE) in the 3′-UTR of the transferrin receptor mRNA and the 5′-UTR of the ferritin mRNA. When iron levels are low, IRBP is free of iron and can, therefore, interact with the IREs. Conversely, when iron levels are high, IRBP binds to iron and then cannot interact with the IRE.
FIGURE 37-9: Iron-mediated regulation of translation of the ferritin and transferrin receptor mRNAs. Reproduced with permission of themedicalbiochemistrypage, LLC.
Protein-Synthesis Inhibitors
Many of the antibiotics utilized for the treatment of bacterial infections as well as certain toxins function through the inhibition of translation. Inhibition can be affected at all stages of translation from initiation to elongation to termination (Table 37-3).
Secreted and Membrane-Associated Proteins
Proteins that are membrane bound or are destined for excretion are synthesized by ribosomes associated with the membranes of the ER (Figure 37-10). The ER associated with ribosomes is termed rough ER (RER). This class of protein all contains an N-terminus termed a signal sequence or signal peptide. The signal peptide is usually 13-36 predominantly hydrophobic residues. The signal peptide is recognized by a multi-protein complex termed the signal recognition particle (SRP). This signal peptide is removed following passage through the ER membrane. The removal of the signal peptide is catalyzed by signal peptidase. Proteins that contain a signal peptide are called preproteins to distinguish them from proproteins. However, some proteins that are destined for secretion are also further proteolyzed following secretion and, therefore contain pro sequences. This class of proteins is termed preproproteins.
FIGURE 37-10: Mechanism of synthesis of membrane bound or secreted proteins. Ribosomes engage the ER membrane through interaction of the signal recognition particle (SRP) in the ribosome with the SRP receptor in the ER membrane. As the protein is synthesized the signal sequence is passed through the ER membrane into the lumen of the ER. After sufficient synthesis the signal peptide is removed by the action of signal peptidase. Synthesis will continue and if the protein is secreted it will end up completely in the lumen of the ER. If the protein is membrane associated a stop transfer motif in the protein will stop the transfer of the protein through the ER membrane. This will become the membrane-spanning domain of the protein. Reproduced with permission of themedicalbiochemistrypage, LLC.
Membrane Targeting by Prenylation
Prenylation refers to the addition of the 15-carbon farnesyl group or the 20-carbon geranylgeranyl group to acceptor proteins, both of which are isoprenoid compounds derived from the cholesterol biosynthetic pathway (see Figure 26-4). Prenylation of a protein allows it to associate with the lipid membrane; thus, this is considered a targeting posttranslational modification. The isoprenoid groups are attached to cysteine residues at the carboxy terminus of proteins (Figure 37-11). A common consensus sequence at the C-terminus of prenylated proteins has been identified and is composed of CAAX, where C is cysteine, A is any aliphatic amino acid (except alanine), and X is the C-terminal amino acid. Following attachment of the prenyl group the carboxylate of the cysteine is methylated in a reaction utilizing S-adenosylmethionine as the methyl donor. At least 60 proteins are known to be prenylated in human tissues.
FIGURE 37-11: Mechanism of protein prenylation. Reproduced with permission of themedicalbiochemistrypage, LLC.
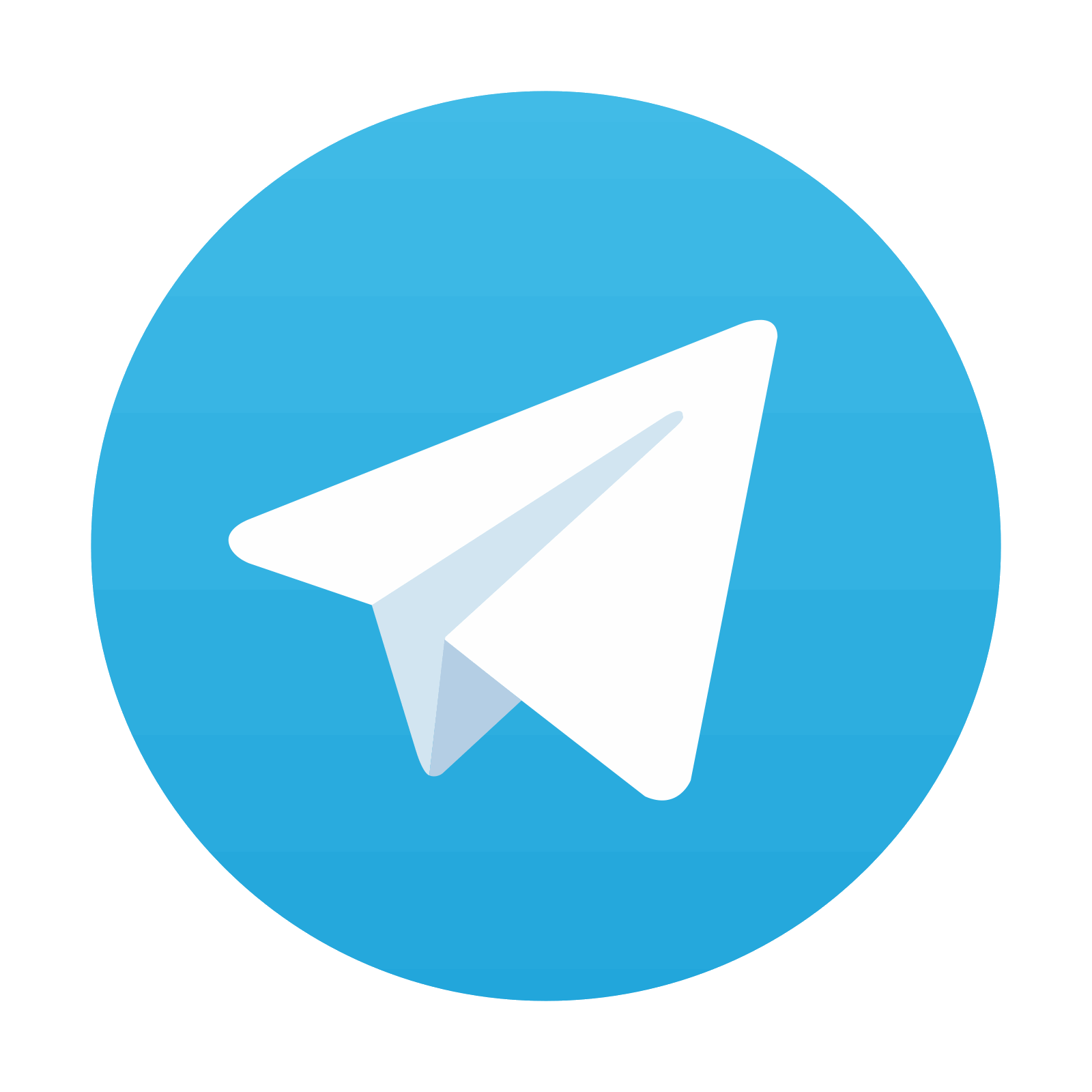
Stay updated, free articles. Join our Telegram channel
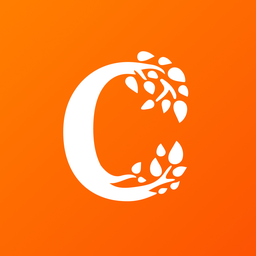
Full access? Get Clinical Tree
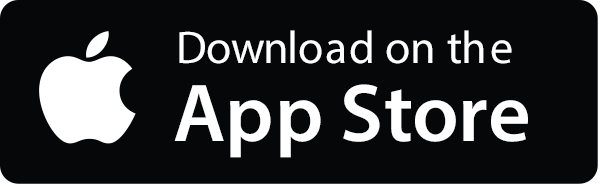
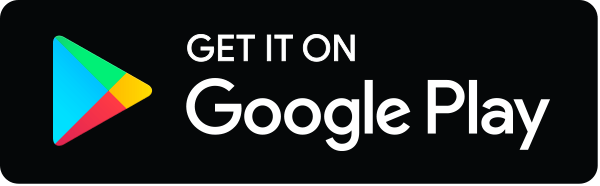