CHAPTER OUTLINE
Major Organ Integration of Metabolism
The Master Metabolic Integrator: AMPK
AMPK and Hypothalamic Functions
Relevance of AMPK to Type 2 Diabetes
Energy Requirements and Reserves
Organ Interrelationships in Well-Fed State
Organ Interrelationships During Fasting or Starvation
High-Yield Terms
Ischemia: refers to a condition caused by restricted blood flow to a tissue, resulting in a shortage of oxygen and glucose needed for continued metabolism
Hypoxia: a condition where a tissue, or the entire body, is restricted or deprived of the flow of oxygen
Pasteur effect: strictly speaking, this refers to the inhibition of bacterial fermentation by oxygen; with respect to eukaryotic metabolism it refers to an increased rate of glycolysis in response to hypoxia
Anorexigenic: causing a suppression of appetite
Orexigenic: causing an increase in appetite
Major Organ Integration of Metabolism
All tissues of the body carry out metabolic processes for survival and growth but not all of the major metabolic pathways are operational at the same time, nor at the same level in all tissues. Changes in nutritional, hormonal, physiological, and pathological status lead to alterations in metabolism in each tissue. In addition, and critical to an understanding of metabolic integration, is that changes in metabolic function in one tissue can, and often do, have potentially profound impacts on the metabolic processes of other tissues.
In the context of overall tissue integration, it is most important to demonstrate how the metabolic processes of the brain, liver, adipose tissue, gastrointestinal tract, and skeletal muscle are interconnected. Within the context of organ integration of metabolism, the pathways that are involved include glycogen synthesis (glycogenesis) and breakdown (glycogenolysis), glycolysis, gluconeogenesis, TCA cycle, lipid synthesis (lipogenesis) and breakdown (lipolysis), lipid oxidation, ketogenesis, amino acid catabolism, urea cycle, protein synthesis, and proteolysis. Each of these processes is most often discussed, as in this text, as isolated metabolic pathways. However, in order to fully appreciate how normal and abnormal physiology and pathology affect the status of the human organism, it is imperative to fully understand their interconnection.
With respect to metabolic integration, the best way to gain an understanding of the processes involved is to examine how these major metabolic pathways respond to everyday feeding and then fasting between meals. Prolonged absence of food results in death within a very short period of time. This fact explains why humans have evolved a complex system of regulatory circuits that were evolutionarily designed to stimulate our desire to seek and consume food. These circuits involve both neuroendocrine (Chapters 43 and 44) and endocrine (Chapters 46, 49, and 50) functions throughout the body. These regulatory pathways allow humans to survive periods of fasting by stimulating energy storage after meal intake and precisely controlling the release of this energy when needed. Unfortunately, humans have not evolved with an equally exquisite means to control the storage of energy. Caloric excess ultimately results in obesity (Chapter 45) and its many associated pathologies including diabetes (Chapter 47), cardiovascular disease (Chapter 48), and cancer (Chapter 52).
Although overall metabolic regulation across multiple tissues as a result of various physiological states involves many different factors (hormones, neuroendocrine factors, enzymes, etc), the most important global regulator is the enzyme, AMP-regulated protein kinase (AMPK). AMPK functions within all tissues to control the level of energy (ATP) consumption and production.
The Master Metabolic Integrator: AMPK
AMPK was first discovered as an activity that inhibited acetyl-CoA carboxylase (ACC) and HMG-CoA reductase (HMGR) and whose activity was induced by increasing AMP concentration. AMPK induces a cascade of events within cells in response to the ever-changing energy charge of the cell. The role of AMPK in regulating cellular energy charge places this enzyme at a central control point in maintaining energy homeostasis. The significance of AMPK activity to overall metabolic integration is that it can also be regulated by physiological stimuli (including nutrients and hormones), independent of the energy charge of the cell.
Once activated, AMPK-mediated phosphorylation events switch cells from active ATP consumption (eg, fatty acid and cholesterol biosynthesis) to active ATP production (eg, fatty acid and glucose oxidation). These events are rapidly initiated and are referred to as short-term regulatory processes. The activation of AMPK also exerts long-term effects at the level of both gene expression and protein synthesis. Other important activities attributable to AMPK are regulation of insulin synthesis and secretion in pancreatic β-cells and modulation of hypothalamic functions involved in the regulation of satiety. How these latter 2 functions impact obesity and diabetes are discussed in Chapters 45 and 46, respectively.
Structure of AMPK
Mammalian AMPK is a trimeric enzyme composed of a catalytic α-subunit and the noncatalytic β- and γ-subunits. There are 2 genes encoding isoforms of both the a- and β-subunits (α1, α2, β1, and β2) and 3 genes encoding isoforms of the γ-subunit (γ1-γ3) allowing for the formation of 12 different isoforms of AMPK. The α2-isoform is the subunit of AMPK found predominantly within skeletal and cardiac muscle, whereas, approximately equal distribution of both the α1 and α2 isoforms are present in hepatic AMPK. Within pancreatic islet β-cells, the α1 isoform predominates, which is also the case for white adipose tissue (WAT). The expression of specific γ-subunits also exhibits tissue specificity with the γ3-subunit found almost exclusively in glycolytic skeletal muscle.
The N-terminal half of the α-subunits contains a typical serine/threonine kinase catalytic domain. Interaction with the β- and γ-subunits occurs via the C-terminal half of the α-subunits. The core of the β-subunits have a glycogen-binding domain (GBD) and the resulting close proximity of AMPK to cellular glycogen stores allows it to rapidly effect changes in glycogen metabolism in response to changes in metabolic demands. The γ-subunits of AMPK bind both regulatory AMP and catalytic ATP and their binding occurs in a mutually exclusive manner. Binding of ATP keeps the activity of AMPK low and when AMP levels rise, the exchange of AMP for ATP results in a 5-fold increase in kinase activity.
High-Yield Concept
Mutations in nucleotide-binding domains of the γ2-subunit (gene symbol PRKAG2) are associated with Wolff-Parkinson-White syndrome and familial hypertrophic cardiomyopathy. An additional inherited disorder associated with mutations in the PRKAG2 gene is a severe cardiac condition called lethal congenital glycogen storage disease of the heart.
Regulation of AMPK
In the presence of AMP, the activity of AMPK is increased approximately 5-fold. However, more importantly is the role of AMP in regulating the level of phosphorylation of AMPK. An increased AMP:ATP ratio leads to a conformational change in the γ-subunit, leading to increased phosphorylation and decreased dephosphorylation of AMPK. The regulatory phosphorylation sites of AMPK reside in the α-subunit. The phosphorylation of AMPK results in activation by at least 100-fold. AMPK is phosphorylated by at least 3 different upstream AMPK kinases (AMPKKs). The activity of AMPK is also regulated by allosteric effectors and by the actions of several hormones, in particular those secreted by adipose tissue (eg, leptin and adiponectin).
As the name implies, AMPK is also regulated by AMP. The effects of AMP are 2-fold: a direct allosteric activation and making AMPK a poorer substrate for dephosphorylation. Because AMP affects both the rate of AMPK phosphorylation in the positive direction and dephosphorylation in the negative direction, the cascade is ultrasensitive. This means that a very small rise in AMP levels can induce a dramatic increase in the activity of AMPK. The activity of adenylate kinase, catalyzing 2ADP to ATP and AMP, ensures that AMPK is highly sensitive to small changes in the intracellular ATP:ADP ratio.
The kinase, LKB1 (also called serine-threonine kinase 11, STK11), which is encoded by the Peutz-Jeghers syndrome tumor suppressor gene (see Chapter 52), is required for activation of AMPK in response to stress. The active LKB1 kinase is actually a complex of 3 proteins: LKB1, Ste20-related adaptor (STRAD), and mouse protein 25 (MO25). LKB1 is widely expressed and is the primary AMPK-regulating kinase.
Calcium-calmodulin–dependent kinase kinase-β (CaMKK-β) phosphorylates and activates AMPK in response to increased intracellular calcium. The distribution of CaMKK-β expression is primarily in the brain. An increased release of intracellular stores of Ca2+ occurs simultaneously with a subsequent demand for ATP. Activation of AMPK, in response to Ca2+ fluxes, thus provides a mechanism for cells to anticipate the increased demand for ATP.
Targets of AMPK
The signaling cascades initiated by the activation of AMPK exert effects on glucose and lipid metabolism, gene expression, and protein synthesis. These effects are most important for regulating integrative metabolism in the liver, skeletal muscle, heart, adipose tissue, and pancreas (Figure 34-1).
FIGURE 34-1: Demonstration of the central role of AMPK in the regulation of metabolism in response to events such as nutrient- or exercise-induced stress. Several of the known physiologic targets for AMPK are included and several pathways as well whose flux is affected by AMPK activation. Arrows indicate positive effects of AMPK, whereas, T-lines indicate inhibitory effects. (See text for definition of enzyme abbreviations.) Reproduced with permission of themedicalbiochemistrypage, LLC.
The uptake, by skeletal muscle, accounts for greater than 70% of the glucose removal from the serum in humans. Therefore, this process is extremely important for overall glucose homeostasis. An important fact related to skeletal muscle glucose uptake is that this process is markedly impaired in individuals with Type 2 diabetes. The uptake of glucose increases dramatically in response to stress (such as ischemia) and exercise and is stimulated by insulin-induced recruitment of glucose transporters to the plasma membrane, primarily GLUT4. Insulin-independent recruitment of GLUT4 also occurs in skeletal muscle in response to contraction (exercise). The ability of AMPK to stimulate GLUT4 translocation to the plasma membrane in skeletal muscle occurs via a mechanism distinct from that stimulated by insulin since together, insulin and AMPK effects are additive. AMPK activation also results in increased expression of the GLUT4 gene. Increased glucose uptake will result in an increase in glycolysis and ATP production.
Under ischemic/hypoxic conditions in the heart, the activation of AMPK leads to the phosphorylation and activation of the kinase activity of PFK-2 (6-phosphofructo-2-kinase). The activation of PFK-2 kinase activity results in increased production of fructose-2,6-bisphosphate (F2,6BP), which in turn potently stimulates PFK-1 activity and increased glycolysis. In liver, the PKA-mediated phosphorylation of PFK-2 results in conversion of the enzyme from a kinase to a phosphatase, opposite the effects exerted by AMPK in the heart. There are 4 isoforms of PFK-2 and neither the liver nor the skeletal muscle isoforms contain the AMPK phosphorylation sites that are found in cardiac PFK-2 (see Chapter 10).
Within skeletal and cardiac muscles, activation of AMPK leads to the phosphorylation and inhibition of both isoforms of acetyl-CoA carboxylase (ACC1 and ACC2). This inhibition results in a drop in the level of malonyl-CoA, which itself is an inhibitor of carnitine palmitoyltransferase I (CPTI). With a drop in the inhibition of CPTI, a concomitant increase in β-oxidation of fatty acids will occur within the mitochondria. An increase in fatty acid oxidation, like increases in glycolysis, will lead to increases in ATP production. In addition to ACC, AMPK has been shown to phosphorylate and thus regulate the activities of HMG-CoA reductase (HMGR); hormone-sensitive lipase (HSL); glycerol-3-phosphate acyltransferase (GPAT); malonyl-CoA decarboxylase (MCD); glycogen synthase (GS), and creatine kinase (CK). Therefore, the effects of AMPK activation are exerted on not only glucose and fatty acid metabolism but overall energy homeostasis, including glycogen metabolism, cholesterol metabolism, and phosphocreatine metabolism.
High-Yield Concept
The ability to activate the kinase activity by phosphorylation of PFK-2 in cardiac tissue in response to ischemic conditions allows these cells to continue to have a source of ATP via anaerobic glycolysis. This phenomenon is recognized as the Pasteur effect: an increased rate of glycolysis in response to hypoxia.
High-Yield Concept
The activity of the inducible form of PFK-2 (iPFK2) is also regulated by AMPK like that of the cardiac isoform. Of pathological significance is the fact that iPFK2 is commonly expressed in many tumor cells and this may allow AMPK to play an important role in protecting tumor cells from hypoxic stress. Indeed, the techniques for depleting AMPK in tumor cells have shown that these cells become sensitized to nutritional stress upon loss of AMPK activity.
High-Yield Concept
When HSL is phosphorylated in response to the activation of PKA, it actively removes fatty acids from adipose tissue triglycerides. In contrast, phosphorylation of HSL by AMPK inhibits its activity. This inhibition may seem paradoxical since the release of stored fatty acids would seem necessary to promote the production of ATP via fatty acid oxidation. This paradigm can be explained if one considers that if the fatty acids that are released from triglycerides are not consumed, they will be recycled back into triglycerides at the expense of ATP consumption. The inhibition of HSL by AMPK is, therefore, likely to be a mechanism to ensure that the rate of fatty acid release does not exceed the rate at which they are utilized either by export or oxidation.
Additional cardiovascular effects exerted by activation of AMPK include phosphorylation and activation of endothelial nitric oxide synthase, eNOS, in cardiac endothelium. AMPK-mediated phosphorylation of eNOS leads to increased activity and consequent NO production and provides a link between metabolic stresses and cardiac function. In platelets, insulin action leads to an increase in eNOS activity as a result of AMPK activity. Activation of NO production in platelets leads to a decrease in thrombin-induced aggregation, thereby, limiting the procoagulant effects of platelet activation. The response of platelets to insulin function clearly indicates why disruption in insulin action is a major contributing factor in the development of the cardiovascular defects in the metabolic syndrome (see Chapter 48).
AMPK activity also leads to changes in the expression of numerous metabolic regulatory genes in the liver and adipose tissue, including the liver isoform of pyruvate kinase (L-PK), fatty acid synthase (FAS), and ACC. Activation of AMPK leads to a reduction in the levels of transcription factors, SREBP (see Chapter 26), and hepatocyte nuclear factor 4α, HNF-4α. Both of these transcription factors are key regulators of the expression of numerous lipogenic enzymes. Of clinical significance is that mutations in HNF-4α are responsible for maturity-onset diabetes of the young, MODY-1 (see Chapter 47).
AMPK activation, in response to hypoxia, exerts negative effects on rates of protein synthesis. Hepatic translation elongation factor 2 (eEF2) is a target for phosphorylation in response to AMPK activation. AMPK phosphorylates and activates the kinase that phosphorylates eEF2 (eEF2K), leading to inhibition of protein synthesis. Another indirect substrate for AMPK that plays a role in protein synthesis is the mammalian target of rapamycin, mTOR (see Chapter 37).
AMPK and Hypothalamic Functions
The activity of AMPK in the hypothalamus is critical in the control of appetite, food intake, and other neuroendocrine functions. The level of active AMPK in the hypothalamus rises during periods of fasting and then decreases in response to refeeding. Pharmacologic activation of hypothalamic AMPK, by the purine nucleotide intermediate AICAR (5-aminoimidazole-4-carboxamide ribotide), leads to increased appetite and a consequent increase in food consumption.
Within the hypothalamus, actions of leptin, GLP-1, and insulin (anorexigenic hormones) result in inhibition of AMPK activation, whereas, actions of adiponectin and ghrelin (orexigenic hormones) result in activation of AMPK activity. Of significance to the tissue-specific distribution of AMPK isoforms, as well as to the differing roles of AMPK in various tissues, the actions of leptin in adipose tissue and liver result in activation of AMPK, while in the heart leptin inhibits AMPK activation as in the hypothalamus. Detailed information on the effects of these peptides and hormones on hypothalamic function is covered in Chapter 44.
Relevance of AMPK to Type 2 Diabetes
Impairment in fuel metabolism occurs in obesity and this impairment is a leading pathogenic factor in the development of Type 2 diabetes. The insulin resistance associated with Type 2 diabetes is most profound at the level of skeletal muscle as this is the primary site of glucose and fatty acid utilization. Therefore, an understanding of how to activate AMPK in skeletal muscle would offer significant pharmacologic benefits in the treatment of Type 2 diabetes. As indicated earlier, it has already been shown that metformin and the thiazolidinedione drugs exert some of their effects via activation of AMPK. In the nonpharmacologic context, activation of AMPK occurs in response to exercise, an activity known to have significant benefit for Type 2 diabetics.
High-Yield Concept
Cells of the gut will see the highest doses of metformin and, therefore, they will experience the greatest level of inhibited complex I activity. This may explain the gastrointestinal side effects (nausea, diarrhea, and anorexia) associated with metformin that limit its utility in many patients.
In skeletal muscle, AMPK phosphorylates the transcription coactivator, PGC1α (peroxisome proliferator–activated receptor-γ coactivator 1α), leading to increased mitochondrial biogenesis and fatty acid oxidation. In addition, AMPK-activated PGC1α results in increased GLUT4 presentation in skeletal muscle cell membranes resulting in increased glucose uptake.
In the liver, AMPK activity enhances fatty acid oxidation via activation of PGC1α in addition to the effects on enzyme activity described earlier. Simultaneously, hepatic cholesterol synthesis is inhibited by AMPK actions at the level of SREBP and HMGR. During fasting AMPK activation results in the inhibition of hepatic gluconeogenesis while simultaneously increasing fatty acid oxidation. The negative effect of AMPK on gluconeogenesis does not involve PGC1α regulation. When fasting, the pancreas releases glucagon which increases hepatic glycogenolysis and gluconeogenesis. The effects of glucagon, on hepatic gluconeogenesis, involve PGC1α and these effects are inhibited by AMPK activity. The effects of hepatic AMPK activation, therefore, override the inductive signals elicited through glucagon.
Several of the current therapies for the treatment of Type 2 diabetes–induced hyperglycemia involve effects on AMPK activity. The thiazolidinedione (TZD) class of drug functions via the activation of peroxisome proliferator–activated receptor γ, PPAR-γ, which is also a target for the action of AMPK. PPAR-γ is primarily expressed in adipose tissue. The TZDs stimulate the expression and release of the adipocyte hormone, adiponectin. Adiponectin stimulates glucose uptake and fatty acid oxidation in skeletal muscle. In addition, adiponectin stimulates fatty acid oxidation in liver while inhibiting expression of gluconeogenic enzymes in this tissue. These responses to adiponectin are exerted via activation of AMPK.
The most widely prescribed Type 2 diabetes drug is metformin. Metformin has a mild inhibitory effect on complex I of oxidative phosphorylation, has antioxidant properties, and activates both glucose 6-phosphate dehydrogenase (G6PDH) and AMPK. The activation of AMPK by metformin is likely related to the inhibitory effects of the drug on complex I of oxidative phosphorylation. This results in a reduction in ATP production and, therefore, an increase in the level of AMP and as a result activation of AMPK.
Energy Requirements and Reserves
The energy needs of any given individual can vary considerably from another due to age, sex, physiological status, and due to various disease states. However, in general, a normal adult of approximately 70 kg (155 lb) requires between 1600 and 2400 calories per day in order to carry out basic metabolic functions. As the level of exertion increases, so too, will the need for caloric intake. The energy reserves in a typical adult are found in the form of carbohydrate (glycogen), fat (triglycerides), and amino acids, primarily as skeletal muscle protein (Table 34-1). As indicated in Table 43-5, the energy contained in fat is on the order of 9 kcal/g and that contained in protein and carbohydrate is approximately 4 kcal/g. These reserves of energy are called upon during fasting and starvation and replenished following feeding. Because glucose can be oxidized for energy, even in the absence of oxygen, it is the most readily utilizable energy source of the body. Fatty acids are released between meals but at high rates only when glycogen reserves are depleted during prolonged fasting. Protein serves as a source of energy because the amino acids can be released and oxidized. It is important to appreciate, though, that protein energy is not readily available like that of glycogen and triglyceride and is, therefore, generally utilized only as a last resort during periods of protracted fasting and starvation.
The 2 major hormones that control the use and storage of energy are the pancreatic hormones, insulin, and glucagon. These 2 hormones serve as counter-regulatory hormones in that they oppose the primary actions of each other. Insulin is released following food intake to prepare the organs to absorb, utilize, and store the energy of the food. Conversely, glucagon is released during fasting in response to falling levels of blood glucose. Glucose levels also fall in stress situations, which also result in release of glucagon. Many other peptide and steroid hormones influence overall energy homeostasis through their actions on various tissues and within the central nervous system. Adipose tissue hormones, such as leptin and adiponectin have important metabolic regulatory functions particularly in the liver and skeletal muscle (see Chapter 45). The adrenal medullary hormones, epinephrine and norepinephrine, and the adrenal cortical steroid hormone, cortisol, each can control energy mobilization in response to various physiological states and due to different types of stress. Gut hormones and neuroendocrine peptides, primarily hypothalamic peptides, control behaviors related to energy homeostasis such as appetite and feeding (see Chapter 44). These same hypothalamic neuropeptides also affect tissue metabolic processes to ensure adequate energy storage and release.
Organ Interrelationships in Well-Fed State
The consumption of food triggers a series of global changes in the disposition of the carbon and nitrogen atoms contained in the carbohydrates, fats, and proteins present in the digested material. The redistribution is determined by the current physiological state and controlled by both endocrine and neuroendocrine functions throughout the body (Figure 34-2). Food intake allows for the replenishment of energy (ATP) used during the period between meals and for the storage of excess carbon to be accessed during the next fast.
FIGURE 34-2: Interrelationships of major organs during well-fed state. Following the consumption of food, the intestines deliver the dietary lipid to the body in the form of chylomicrons via the lymphatic system to the left subclavian vein. Dietary glucose and amino acids enter the circulation via the superior mesenteric vein to the portal vein that enters the liver. Food intake stimulates pancreatic secretion of insulin, which enters the portal vein and then the overall circulation of the body where it activates various processes in tissues such as adipose tissue and skeletal muscle. Dietary fatty acids and fatty acids from the liver (in VLDL) are taken up by numerous tissues, where they are oxidized and/or stored as triglycerides. In the well-fed state, insulin increases lipoprotein lipase (LPL) density on endothelial cells, promoting fatty acid uptake and GLUT4 density in adipose tissue and skeletal muscle, promoting glucose uptake. Reproduced with permission of themedicalbiochemistrypage, LLC.
The primary hormonal response to the intake of food is the release of insulin from the pancreas. The action of insulin, on its primary target tissues, skeletal muscle, and adipose tissue, is to ensure that glucose is readily absorbed from the blood and that the enzymes necessary for utilization and storage of the incoming carbon atoms (carbohydrates, fats, amino acids) are fully active.
Following consumption of food, the stomach and intestines digest the complex polymeric molecules into smaller monomeric units so that fatty acids, monoglycerides, amino acids, and monosaccharides can be absorbed. These processes are discussed in detail in Chapter 43. Within the intestines, glucose, amino acids, and fats can be utilized for the energy needs of this tissue. The primary source of carbon for ATP generation in the intestines is the absorbed amino acids.
Free fatty acids (FFAs) are not transported to the portal circulation from the intestines. They are first incorporated into triglycerides and phospholipids and then packaged into the lipoprotein particles called chylomicrons (see Chapter 28) and delivered to the lymphatic system. The intestinal lymphatics feed into the thoracic duct and then into the left subclavian vein for distribution throughout the body. The action of lipoprotein lipase (LPL) on endothelial cells of the vasculature allows dietary fatty acids to be absorbed by the tissues. Skeletal and cardiac muscle prefer fatty acid oxidation as a means to acquire ATP energy. Adipose tissue will incorporate the fatty acids into triglycerides for storage and future release during periods of fasting and starvation.
The primary dietary monosaccharides are glucose, fructose, and galactose. When delivered to the portal circulation, the high Km of hepatic glucokinase ensures that the vast majority of the glucose passes through this organ to be utilized by other tissues. The brain is almost completely dependent on glucose oxidation for energy, so delivery of this carbohydrate to the brain is especially important during refeeding. In addition, erythrocytes and kidney medullary cells are absolutely dependent upon glucose oxidation for ATP production. Insulin released in response to food intake stimulates the uptake of glucose by skeletal muscle and adipose tissue. Within muscle cells, the glucose is stored as glycogen or oxidized for ATP production. Some of the ATP is used to phosphorylate creatine, generating creatine phosphate that is a rapidly utilizable source of phosphate for skeletal muscle ATP generation. Adipose tissue carries out glycolysis for both ATP production as well as diversion of the glucose carbons into the glycerol backbone of triglycerides. This latter function results from glycolytic DHAP being converted to glycerol 3-phosphate via the action of glycerol-3-phosphate dehydrogenase. Given that adipocytes do not express glycerol kinase, the use of DHAP demonstrates the absolute requirement for glycolysis in adipose tissue for fat storage as triglycerides. As the level of glucose rises in the blood, the liver will begin to trap some for use and storage in this tissue through the increased activity of glucokinase. The release of insulin alters the metabolic processes of the liver so that the glucose is stored as glycogen or oxidized to pyruvate. The pyruvate can either be reduced to lactate or oxidized to acetyl-CoA. The resulting acetyl-CoA is then utilized for fatty acid and cholesterol synthesis or completely oxidized to CO2 and water in the TCA cycle.
In the well-fed state, lactate produced by glycolysis in erythrocytes, skeletal muscle, and other tissues is taken up by the liver and oxidized to pyruvate. The pyruvate generated in this way experiences the same fate as hepatic glycolysis-derived pyruvate; complete oxidation or the resulting acetyl-CoA diverted to fat and cholesterol synthesis. Due to the hormonal states following feeding, high insulin and low glucagon, the liver is not actively carrying out gluconeogenesis, so little, if any, lactate is utilized in this latter pathway. Therefore, the Cori cycle is minimally active in the well-fed state.
Dietary amino acids delivered to the portal circulation generally pass through the liver as a consequence of the high Km of amino acid catabolic enzymes. This ensures that dietary amino acid, particularly essential amino acids, can be acquired by peripheral tissue for oxidation and/or protein synthesis. When oxidized in the liver, or for that matter in other tissues, the waste nitrogen is incorporated into urea and excreted. Within the liver, as well as peripheral tissues, the Km of the aminoacyl-tRNA synthetases are low, ensuring that protein synthesis is able to occur, especially in the well-fed state when the load of amino acids is high.
As chylomicrons move through the vasculature and are acted upon by LPL, they lose fatty acids and become chylomicron remnants. The remnants are taken up by the liver and degraded in lysosomes. The released fatty acids are re-esterified to glycerol 3-phosphate (derived from glycolysis) and the triglycerides are packaged into VLDL. The VLDLs are then released to the circulation where the action of LPL exerts the same effects on these lipoprotein particles as in the case of chylomicrons.
Organ Interrelationships During Fasting or Starvation
The primary, whole-body response to the periods between feeding occurs due to the shift in pancreatic hormone secretion. These changes in hormone release result primarily from falling serum glucose levels. As serum glucose levels drop, the rate of insulin secretion falls and the rate of glucagon secretion increases. The major responses of the tissues to glucagon occur at the level of adipose tissue and the liver, triggering release of energy and conversion of carbon into glucose. Although the kidneys and the small intestines can contribute to overall endogenous glucose production, the liver is the most important organ controlling blood glucose levels, particularly during periods of fasting and starvation (Figure 34-3).
FIGURE 34-3: Interrelationships of major organs during fasting state. During fasting the blood glucose level falls, prompting the pancreas to secrete glucagon which stimulates the liver to undertake gluconeogenesis and glycogenolysis to provide glucose to the blood. Glucagon also stimulates adipose tissue to release fatty acids so that tissues such as the heart and skeletal muscles have adequate energy. The fatty acids released from adipose tissue are also oxidized by the liver to provide the energy necessary for gluconeogenesis. In addition, hepatic fatty acid oxidation provides the major source of acetyl-CoA for ketone synthesis, which is necessary to provide the brain with an energy source during periods of glucose restriction. In addition to gluconeogenesis in the liver, the kidneys and the duodenum carry out gluconeogenesis to contribute to endogenous glucose production during fasting. Reproduced with permission of themedicalbiochemistrypage, LLC.
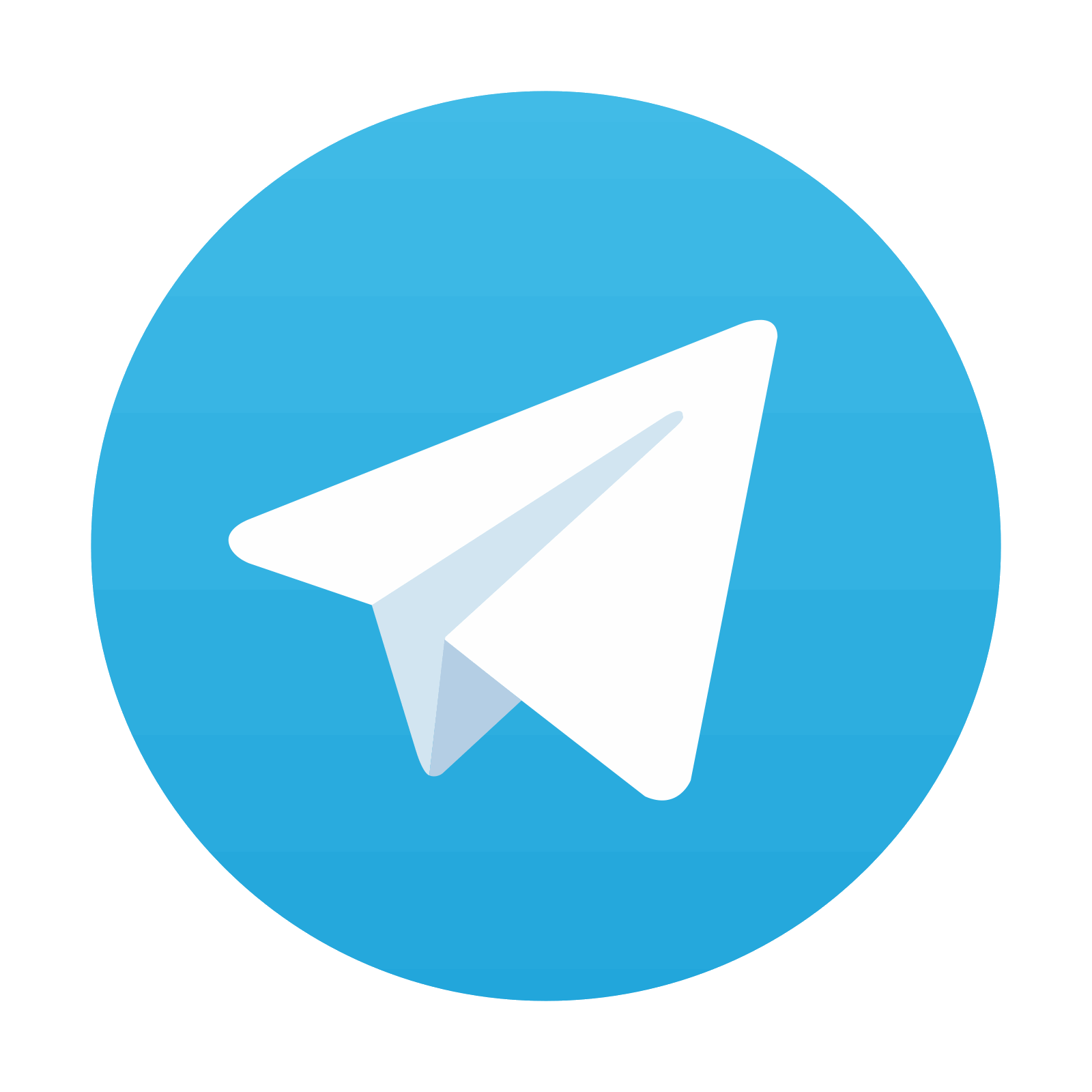
Stay updated, free articles. Join our Telegram channel
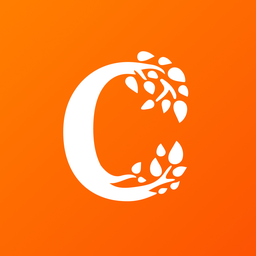
Full access? Get Clinical Tree
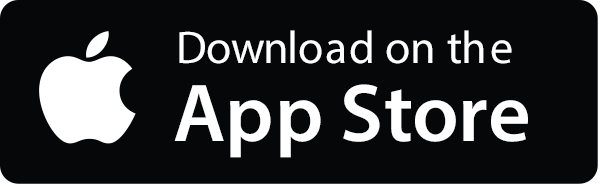
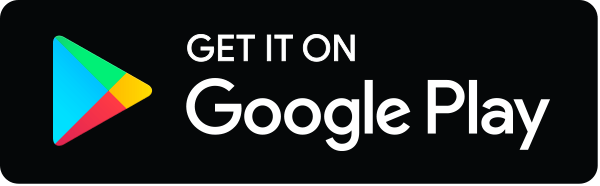