High-Yield Terms
Porphyrin: a group of aromatic organic compounds composed of 4 modified pyrrole subunits interconnected at their α-carbon atoms via methine bridges
Porphyria: any of a group of rare inherited or acquired disorders of enzymes involved in the production of the porphyrins and heme
Hemin: protoporphyrin IX containing a ferric (Fe3+) iron, as opposed to heme which contains ferrous (Fe2+) iron
Reticuloendothelial cells: a collective term for cells of the immune system that primarily comprises macrophages and monocytes but may include all phagocytic cells
Bilirubin: open chain yellowish breakdown product of heme catabolism
Kernicterus: bilirubin-induced brain dysfunction, also called bilirubin encephalopathy
Sideroblastic anemia: a disease in which the bone marrow produces ringed sideroblasts rather than healthy erythrocytes, sideroblasts are abnormal nucleated erythroblasts with granules of iron in perinuclear mitochondria
Porphyrins and Heme
The porphyrins (Figure 33-1) are a group of aromatic organic compounds composed of 4 modified pyrrole subunits interconnected at their α-carbon atoms via methine bridges (=CH–). These compounds are highly conjugated systems and as such they typically have very intense absorption bands in the visible region and may be deeply colored. Indeed, the name porphyrin comes from a Greek word for purple. In humans, the most clinically significant porphyrins are the hemes; heme a, heme b, and heme c. Heme b is called protoporphyrin IX and it contains 4 methyl, 2 vinyl, and 2 propionic acid substituents. Heme b is the prosthetic group that binds oxygen in erythrocytes and is the pigment that induces the reddish color of blood. Heme a and heme c are prosthetic groups of several cytochromes, particularly of the electron transport chain (Chapter 17). Many additional proteins contain a heme prosthetic group with the largest family of such proteins being the cytochrome P450 (CYP) enzymes, many of which are involved in xenobiotic metabolism in the liver.
FIGURE 33-1: The porphyrin molecule. Rings are labeled I, II, III, and IV. Substituent positions on the rings are labeled 1, 2, 3, 4, 5, 6, 7, and 8. The methyne bridges (=HC—) are labeled α, β, γ, and δ. The numbering system used is that of Hans Fischer. Murray RK, Bender DA, Botham KM, Kennelly PJ, Rodwell VW, Weil PA. Harper’s Illustrated Biochemistry, 29th ed. New York: McGraw-Hill; 2012.
Synthesis of Porphyrins and Heme
The first reaction in heme biosynthesis takes place in the mitochondrion and involves the condensation of 1 glycine and 1 succinyl-CoA by the pyridoxal phosphate-containing enzyme, δ-aminolevulinic acid synthase (ALAS) (Figure 33-2). Delta-aminolevulinic acid (ALA) is also called 5-aminolevulinic acid. This reaction is both the rate-limiting reaction of heme biosynthesis and the most highly regulated reaction. There are 2 forms of ALAS. ALAS1 is considered a house-keeping gene and is expressed in all cells. ALAS2 is an erythroid-specific form of the enzyme and is expressed only in fetal liver and adult bone marrow.
FIGURE 33-2: Biosynthesis of porphobilinogen. ALA synthase occurs in the mitochondria, whereas ALA dehydratase is present in the cytosol. Murray RK, Bender DA, Botham KM, Kennelly PJ, Rodwell VW, Weil PA. Harper’s Illustrated Biochemistry, 29th ed. New York: McGraw-Hill; 2012.
Mitochondrial ALA is transported to the cytosol, where ALA dehydratase (also called porphobilinogen synthase) dimerizes 2 molecules of ALA to produce the pyrrole-ring compound porphobilinogen (see Figure 33-2). The next step in the pathway involves the head-to-tail condensation of 4 molecules of porphobilinogen to produce the linear tetrapyrrole intermediate, hydroxymethylbilane catalyzed by porphobilinogen deaminase, PBG deaminase (Figure 33-3). PBG deaminase is also called hydroxymethylbilane synthase or uroporphyrinogen I synthase. Hydroxymethylbilane has 2 main fates. The most important is regulated, enzymatic conversion to uroporphyrinogen III, the next intermediate on the path to heme. This step is mediated by a holoenzyme comprised of uroporphyrinogen synthase plus a protein known as uroporphyrinogen III cosynthase. Hydroxymethylbilane can also non-enzymatically cyclize forming uroporphyrinogen I (see Figure 33-3).
FIGURE 33-3: Conversion of porphobilinogen to uroporphyrinogens. Uroporphyrinogen synthase I is also called porphobilinogen (PBG) deaminase or hydroxymethylbilane (HMB) synthase. Murray RK, Bender DA, Botham KM, Kennelly PJ, Rodwell VW, Weil PA. Harper’s Illustrated Biochemistry, 29th ed. New York: McGraw-Hill; 2012.
In the cytosol, the acetate substituents of uroporphyrinogen (normal uroporphyrinogen III or abnormal uroporphyrinogen I) are all decarboxylated by the enzyme uroporphyrinogen decarboxylase (Figure 33-4). The resultant products have methyl groups in place of acetate and are known as coproporphyrinogens, with coproporphyrinogen III being the important normal intermediate in heme synthesis. Coproporphyrinogen III is transported back into the mitochondria, where 2 propionate residues are decarboxylated, yielding vinyl substituents on the 2 pyrrole rings. The colorless product is protoporphyrinogen IX. In the mitochondrion, protoporphyrinogen IX is converted to protoporphyrin IX by protoporphyrinogen IX oxidase. The oxidase reaction requires molecular oxygen and results in the loss of 6 protons and 6 electrons, yielding a completely conjugated ring system, which is responsible for the characteristic red color to hemes. The final reaction in heme synthesis also takes place in the mitochondrion and involves the insertion of the iron atom into the ring system generating heme b. The enzyme catalyzing this reaction is known as ferrochelatase.
FIGURE 33-4: Steps in the biosynthesis of the porphyrin derivatives from porphobilinogen. Uroporphyrinogen I synthase is also called porphobilinogen deaminase or hydroxymethylbilane synthase. Murray RK, Bender DA, Botham KM, Kennelly PJ, Rodwell VW, Weil PA. Harper’s Illustrated Biochemistry, 29th ed. New York: McGraw-Hill; 2012.
Clinical problems associated with heme metabolism are of 2 types. Disorders that arise from defects in the enzymes of heme biosynthesis are termed the porphyrias and cause elevations in the levels of intermediates in heme synthesis in the serum and urine. Inherited disorders in bilirubin metabolism lead to hyperbilirubinemia which is the root cause of jaundice.
High-Yield Concept
Deficiencies of ALAS2 result in a disorder called X-linked sideroblastic anemia, XLSA. Sideroblasts are erythroblasts with non-heme iron–containing organelles, called siderosomes. XLSA has also been called congenital sideroblastic anemia, hereditary sideroblastic anemia, hereditary iron-loading anemia, X-linked hypochromic anemia, hereditary hypochromic anemia, and hereditary anemia.
High-Yield Concept
The enzymes ferrochelatase, ALA synthase, and ALA dehydratase are highly sensitive to inhibition by heavy metal poisoning. Indeed, a characteristic of lead poisoning is an increase in ALA in the circulation in the absence of an increase in porphobilinogen.
Regulation of Heme Biosynthesis
Although heme is synthesized in virtually all tissues, the principal sites of synthesis are erythroid cells (≈85%) and hepatocytes. The differences in these 2 tissues and their needs for heme result in quite different mechanisms for regulation of heme biosynthesis.
In hepatocytes, heme is required for incorporation into the cytochromes, in particular, the P450 class of cytochromes that are important for detoxification. In addition numerous cytochromes of the oxidative-phosphorylation pathway contain heme. The rate-limiting step in hepatic heme biosynthesis occurs at the ALAS catalyzed step, which represents the committed step in heme synthesis. The Fe3+ oxidation product of heme is termed hemin. Hemin acts as a feedback inhibitor of ALAS. Hemin also inhibits the synthesis of ALAS and inhibits its transport from the cytosol into the mitochondria.
In erythroid cells all of the heme is synthesized for incorporation into hemoglobin and occurs only upon differentiation when synthesis of hemoglobin proceeds. In mature erythrocytes both heme and hemoglobin syntheses cease. The heme and hemoglobin must, therefore, survive for the life of the erythrocyte (normally this is around 120 days). In reticulocytes, heme stimulates protein synthesis (see Chapter 37).
The heme oxygenase reaction is the only one that is known to produce CO. Most of the CO is excreted through the lungs, with the result that the CO content of expired air is a direct measure of the activity of heme oxygenase in an individual.
Heme Catabolism
The largest repository of heme in the human body is in erythrocytes. The daily turnover of these cells results in the release of about 6 g/d of hemoglobin. In the catabolism of the heme from hemoglobin the porphyrin ring is hydrophobic and must be solubilized for it to be excreted. In addition, the iron must be conserved for new heme synthesis. Senescent erythrocytes, and heme from other sources, are engulfed by cells of the reticuloendothelial system. The globin proteins are recycled or degraded into amino acids which are, in turn, recycled or catabolized as needed.
Heme is oxidized, with the heme ring being opened by the endoplasmic reticulum enzyme, heme oxygenase (Figure 33-5). This oxidation step requires heme as a substrate, and any hemin (Fe3+) is reduced to heme (Fe2+) first. The oxidation produces the linear tetrapyrrole biliverdin, ferric iron (Fe3+), and carbon monoxide (CO).
FIGURE 33-5: Heme catabolism initiated by the action of heme oxygenase. The heme ring is cleaved by heme oxygenase yielding biliverdin. Biliverdin is then converted to bilirubin via the action of bilverdin reductase. The side groups on bilverdin and bilirubin are abbreviated: M, methyl; V, vinyl, P, propyl. Reproduced with permission of themedicalbiochemistrypage, LLC.
The next reaction is catalyzed by biliverdin reductase, producing bilirubin. Bilirubin is significantly less conjugated than biliverdin causing a change in the color of the molecule from blue-green (biliverdin) to yellow-red (bilirubin). The latter catabolic processes are responsible for the progressive changes in the color of a hematoma, or bruise, in which the damaged tissue changes its color from an initial dark blue to a red-yellow and finally to a yellow color before all the pigment is transported out of the affected tissue. Peripherally arising bilirubin is transported to the liver in association with albumin, where the remaining catabolic reactions take place.
In hepatocytes, bilirubin-UDP-glucuronyltransferase (bilirubin-UGT) adds 2 equivalents of glucuronic acid to bilirubin to produce the more water-soluble bilirubin diglucuronide derivative (Figure 33-6). Bilirubin-UGT is encoded by the UGT1A gene, and several UGT1A enzymes including bilirubin-UGT (identified as UGT1A1) are encoded by the UGT1A gene complex. The increased water solubility facilitates its excretion with bile. In the intestines, bilirubin and its catabolic products, generated by bacterial metabolism, are collectively known as the bile pigments. The overall process of bilirubin transport, conjugation, and excretion in the bile is summarized in Figure 33-7. The sites in this process where defects are known to cause various disorders of bilirubin metabolism resulting in hyperbilirubinemias are also indicated.
FIGURE 33-6: Structure of bilirubin diglucuronide (conjugated, “direct-reacting” bilirubin). Glucuronic acid is attached via ester linkage to the two propionic acid groups of bilirubin to form an acylglucuronide. Murray RK, Bender DA, Botham KM, Kennelly PJ, Rodwell VW, Weil PA. Harper’s Illustrated Biochemistry, 29th ed. New York: McGraw-Hill; 2012.
FIGURE 33-7: Diagrammatic representation of the three major processes (uptake, conjugation, and secretion) involved in the transfer of bilirubin from blood to bile. Certain proteins of hepatocytes, such as ligandin (a member of the glutathione S-transferase family of enzymes) and Y protein, bind intracellular bilirubin and may prevent its efflux into the blood stream. The process affected in a number of conditions causing jaundice is also shown. Murray RK, Bender DA, Botham KM, Kennelly PJ, Rodwell VW, Weil PA. Harper’s Illustrated Biochemistry, 29th ed. New York: McGraw-Hill; 2012.
Abnormalities in Heme Metabolism
Excess circulation and accumulation of bilirubin (hyperbilirubinemia) results jaundice. There are 2 major classes of hyperbilirubinemias: unconjugated and conjugated (Clinical Box 33-1). Because unconjugated bilirubin is not water soluble and therefore much more toxic, the clinical symptoms of unconjugated hyperbilirubinemias can be severe and even fatal. Several inherited disorders in bilirubin metabolism have been identified. Gilbert syndrome (Clinical Box 33-2) and the Crigler-Najjar syndromes (Clinical Box 33-3) result from predominantly unconjugated hyperbilirubinemia. Dubin-Johnson syndrome and Rotor syndrome result from conjugated hyperbilirubinemia.
CLINICAL BOX 33-1: HYPERBILIRUBINEMIA CLASSIFICATIONS
There are 2 major classes of hyperbilirubinemias: unconjugated and conjugated. Bilirubin levels are measured in the serum by an assay utilizing Ehrlich diazo reagent and results in the formation of an azobilirubin product. Conjugated bilirubin does not require addition of alcohol to promote the azotization reaction, and thus this is referred to as measurement of direct bilirubin. The reaction with unconjugated bilirubin requires the addition of alcohol, and thus is referred to as the measurement of indirect bilirubin. Normal bilirubin measurements are 0.3 to 1.2 mg/dL for total (indirect + direct). Direct type bilirubin does not exist in the plasma; however, a small portion of indirect type bilirubin may present as direct reacting type and thus the serum measurement may show a direct bilirubin, but this is never above 0.3mg/dL in a normal individual. The mildest form of this condition is jaundice which is caused by a yellow-orange discoloration of the tissues and is most easily visible as icteric discoloration in the sclera of the eyes. Bilirubin toxicity (bilirubin encephalopathy) can be life threatening in neonates. Bilirubin encephalopathy is characterized by yellow discoloration of the basal ganglia in babies with intense jaundice and is termed kernicterus. Any increase in plasma bilirubin above 20 mg/dL is considered dangerous in neonates. However, individual differences in bilirubin sensitivity can result in kernicterus at lower bilirubin levels. Kernicterus occurs in infants with severe unconjugated hyperbilirubinemia and in young adults with high serum levels of unconjugated bilirubin, with the latter the result of inherited deficiencies in bilirubin-UGT. Bilirubin has been shown to inhibit DNA synthesis, uncouple oxidative phosphorylation, and inhibit ATPase activity in brain mitochondria. Bilirubin also inhibits a variety of different classes of enzymes including dehydrogenases, electron transport proteins, hydrolases, and enzymes of RNA synthesis, protein synthesis, and carbohydrate metabolism. All of these toxic effects of bilirubin are reversed by binding to albumin. In fact, albumin plays a vital role in the disposition of bilirubin in the body by keeping the compound in solution and transporting it from its sites of production (primarily bone marrow and spleen) to its site of excretion which is the liver.
CLINICAL BOX 33-2: GILBERT SYNDROME
Gilbert syndrome results from mutations in the TATA-box of the promoter region upstream of exon 1 in the UGT1A gene which results in reduced levels of expression of a normal bilirubin-UGT enzyme. The normal sequence of the UGT1A TATA-box is A(TA)6TAA, whereas in Gilbert syndrome individuals it is A(TA)7TAA. Gilbert syndrome is also referred to as constitutional hepatic dysfunction and familial nonhemolytic jaundice. The syndrome is characterized by mild chronic, unconjugated hyperbilirubinemia. Almost all afflicted individuals have a degree of icteric discoloration in the eyes typical of jaundice. Serum bilirubin levels in Gilbert syndrome patients are usually less than 3 mg/dL. Many patients manifest with fatigue and abdominal discomfort, symptoms that are ascribed to anxiety, but are not due to bilirubin metabolism. Expression of the Gilbert phenotype requires a relatively high level of bilirubin production. This is evident from the fact that persons homozygous for the UGT1A TATA-box mutation do not exhibit hyperbilirubinemia. Presentation of Gilbert syndrome symptoms also occurs more frequently in men than in women because the production of bilirubin is higher in males.
CLINICAL BOX 33-3: CRIGLER-NAJJAR SYNDROMES
There are two forms of Crigler-Najjar syndrome: type I results from mutations in the bilirubin-UGT gene that result in complete loss of enzyme activity, whereas type II is the result of mutations that cause incomplete loss of enzyme activity.
Type I: In patients with very high levels of unconjugated bilirubin, but with normal liver function tests, Crigler-Najjar syndrome type I is indicated. Almost all afflicted infants manifest with severe nonhemolytic icterus within the first few days of life. The jaundice in these patients is characterized by increased concentrations of indirect-reacting bilirubin in the plasma. The advent of phototherapy has allowed for greater survival in type I patients; in fact without this therapy infants will succumb to kernicterus by the age of 15 months. Use of phototherapy and intermittent plasmapheresis has allowed many type I infants to survive until puberty without significant brain damage. The risk for kernicterus persists following puberty, however, because phototherapy is less effective at this age. Liver transplantation is considered the only definitive treatment for type I Crigler-Najjar syndrome.
Type II: The clinical manifestations of type II disease are similar to those of type I except that serum bilirubin levels are much lower, generally below 20 mg/dL. The prognosis for type II patients is also much better than for type I patients. Induction of bilirubin-UGT by drugs such as phenobarbitol can lead to reductions in serum bilirubin levels in type II patients. Given that type I Crigler-Najjar results from complete loss of functional bilirubin-UGT it is not surprising that phenobarbitol has no effect in those patients. Type II Crigler-Najar syndrome was first described by I.M. Arias and thus, this form of the disease is also sometimes referred to as Arias syndrome.
The porphyrias are both inherited and acquired disorders in heme synthesis. These disorders are classified as either erythroid or hepatic, depending upon the principal site of expression of the enzyme defect. Eight different porphyrias have been classified encompassing defects in each of the enzymes of heme synthesis (Table 33-1). The most commonly occurring hepatic porphyria is acute intermittent porphyria (AIP) (Clinical Box 33-4), which is caused by a defect in PBG deaminase. This enzyme is also called hydroxymethylbilane synthase or rarely uroporphyrinogen I synthase. All of the porphyrias lead to excretion of heme biosynthetic by-products that turn the urine red and when deposited in the teeth turn them reddish brown. Accumulation of these by-products in the skin renders it extremely sensitive to sunlight causing ulceration and disfiguring scars. Increased hair growth (hypertrichosis) is also a symptom of the porphyrias leading to appearance of fine hairs over the entire face and on the extremities. This latter symptom lends to the description of “werewolf syndrome” in many porphyria patients.
CLINICAL BOX 33-4: ACUTE INTERMITTENT PORPHYRIA, AIP
Acute intermittent porphyria (AIP) is an autosomal dominant disorder that results from defects in PBG deaminase (PBGD) resulting in 50% of normal activity. AIP is classified as an acute hepatic porphyria and is the most common hepatic type porphyria. AIP is prevalent in Swedish populations, particularly northern Swedes where the frequency approaches 100 cases per 100,000 population. Because of this prevalence, AIP is also known as Swedish porphyria. Using immunological assays for the presence of PBGD activity, mutations in the PBGD gene have been classified into 3 distinct categories. Type I mutations result in about 50% detectable PBGD protein and enzyme activity. These mutations are referred to as CRIM-negative mutations where CRIM means “cross-reactive immunologic material.” Type I mutations represent the largest percentage of mutations in AIP patients, consisting of approximately 85% of mutations. Type II mutations are also CRIM-negative and result in absence of the house-keeping form of the enzyme but normal amounts of the erythroid-specific isozyme. These mutations are found in less than 5% of AIP patients. Type III mutations are CRIM-positive and result in decreased PBGD activity but do not alter the stability of the mutant enzyme. The clinical manifestations of AIP are related to the visceral, autonomic, peripheral, and central nervous system involvement of the disease. In fact almost all of the symptoms of AIP result from neurological dysfunction; however, the exact mechanism leading to neural cell damage is not clearly understood. The pain associated with the neurological dysfunction can be so severe as to require treatment with opiates. The symptoms of AIP result from a dramatic increase in the production and excretion of porphyrin precursors. A reduced ability to synthesize heme in a state of increased demand results in loss of heme-mediated control of the rate-limiting enzyme of heme synthesis, ALAS1. In this circumstance, unregulated ALAS1 results in large increases in synthesis of ALA. The large increases in ALA drive the ALA dehydratase reaction to produce large amounts of PBG. With the block in heme synthesis at the PBGD reaction the resulting increases in ALA and PBG synthesis leads to marked increases in urinary excretion of both compounds resulting in deep red urine, a hallmark of AIP. Clinical manifestation in AIP becomes apparent in the event of an increased demand for hepatic heme production. This most often occurs due to drug exposure or some other precipitating factor such as an infection. In fact, an infection is the leading cause of attacks of acute porphyria. If a drug is the cause of an attack its use should be discontinued immediately. The neurovisceral signs and symptoms of AIP are nonspecific and highly variable resulting in patients being diagnosed with an unrelated illness. Because certain factors such as hormonal or nutritional influences or drug interactions can affect the clinical manifestation of AIP, as well as other hepatic porphyrias, these diseases are referred to as ecogenetic or pharmacogenetic disorders. Numerous drugs are known to lead to acute attacks of porphyria, in particular barbiturates. Other drugs include ACE inhibitors and calcium channel blockers, the sulfonylureas used by type 2 diabetics, and the sulfonamide class of antibiotic. The most common symptom (occurring in 85%-95% of cases) during an acute attack is diffuse, poorly localized abdominal pain which can be quite severe. The abdominal pain is usually accompanied by constipation, nausea, and vomiting. The most common physical sign that is observed in up to 80% of AIP cases is tachycardia which may be due to sympathetic nervous system overactivity. Additional symptoms of AIP are pain in the limbs, head, neck, or chest; muscle weakness; and sensory loss. Often, without any precipitating influences, AIP remains latent and there may be no family history of the disease. The symptoms of AIP are almost never observed in prepubescent children and are seen more frequently in women than in men. Treating the symptoms of AIP involves a high-carbohydrate diet and during a severe attack, an infusion of 10% glucose is highly recommended. Panhematin, a form of alkaline heme, is used to treat attacks of porphyria as it acts like heme to inhibit ALAS, thereby reducing the production of ALA and subsequently PBG.
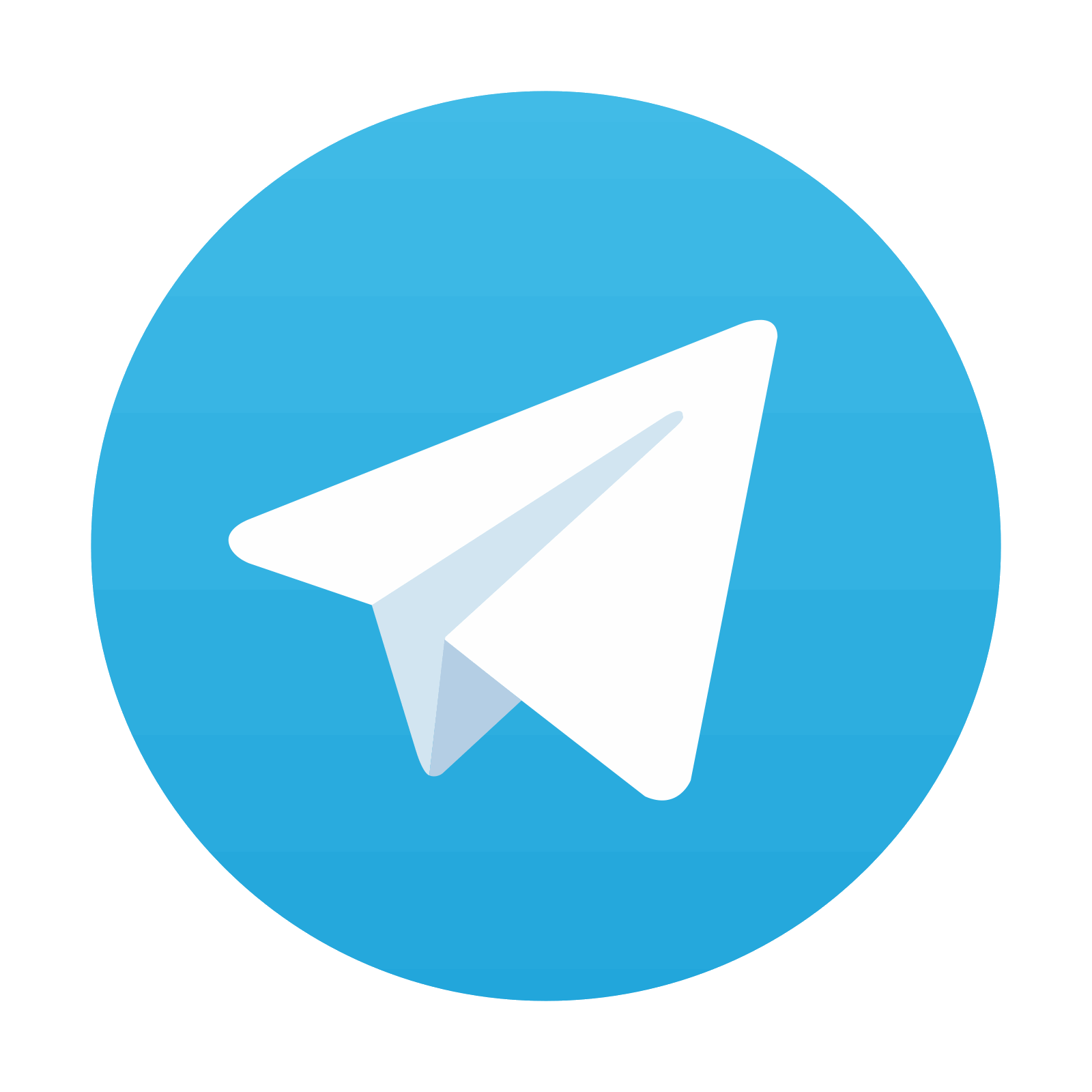
Stay updated, free articles. Join our Telegram channel
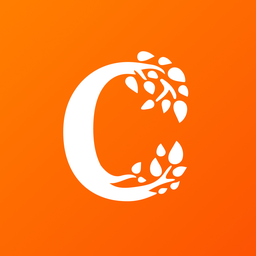
Full access? Get Clinical Tree
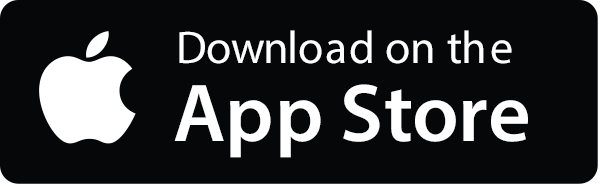
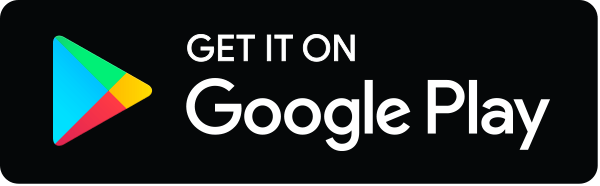